Microfluidics: The fur-free way towards personalised medicine in cancer therapy
Posted: 17 March 2016 | Amy Dawson (University of Hull), John Greenman (University of Hull), Ruth Bower (University of Hull), Victoria Green (University of Hull) | 1 comment
Microfluidic technology has great potential for complementing and, in some instances, replacing the use of animal models in the testing of medicines and in developing personalised treatments for cancer patients.
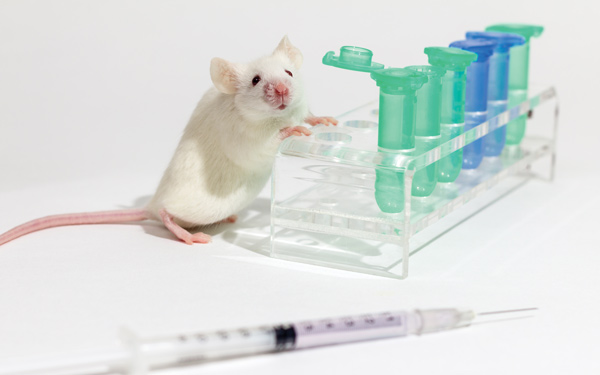
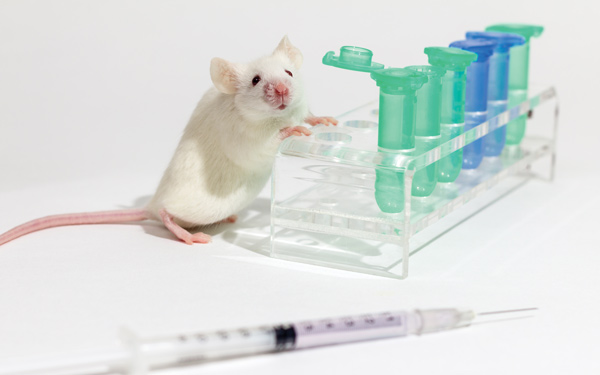
The maintenance of tissue in an in vivo-like state provides a platform upon which normal and diseased tissue biology can be investigated in a novel way. This review describes the use of microfluidic technology for the maintenance of tissue samples ex vivo and the current state of play for the use of this technology in the replacement of animal models, with a focus on cancer.
Currently, the majority of cancer treatments rely on a coordinated multi-faceted approach, utilising evidence gathered from large-scale animal studies and human clinical trials, in order to determine an optimal treatment regimen. Unfortunately, the efficacy of many therapies is relatively poor with some causing severe side effects and many tumours having five year survival rates not exceeding 50%1-2. It is becoming increasingly clear that the variability in disease patterns and cancer response is due to the unique genetic background and immune response of each patient3-5. Hence the best way to deal with individual tumours is to optimise the treatment on a person-by-person basis; this is not easily done with current methodologies6-7.
Traditionally, development of treatments for diseases such as cancer use simple in vitro cell culture models and/or animal models to understand the complex patho-physiology of the disease6 .
Cell culture models typically use a single cell type grown in a monolayer, whereas the majority of cells in vivo grow in three dimensional (3D) structures consisting of many different cell types in a unique spatial arrangement, interweaved with extracellular matrix (ECM). It is the sum of all these interactions between neigh bouring cells and the ECM that guides developmental processes central for function. Although the fabrication of 3D spheroids constructed from different cell types is designed to try and mimic these interactions, the complex in vivo nature is still not matched.
Approaches using xenograft models by Stebbing et al12 have shown promising data towards personalised cancer treatment: resected tumour is divided, grafted subcutaneously into immunocompromised mice, propagated and passaged into further generations to provide multiple animals carrying the same tumour. These can then be tested with a variety of drugs alone or in combination to establish which works most effectively. However, the procedures are lengthy with the growing process taking up to six months, potentially leading to a delay in patients recieving the best treatment, and the mouse avatars cost tens of thousands of dollars to generate and maintain with graft success rates of less than 100%12-13. Furthermore, the approach uses a relatively large number of animals which comes at a time when society is keen to reduce the numbers of animals used in research. Finally, there is the obvious limitation in that substantial differences do exist in the systems and mechanisms between humans and mice.
Microfluidics is a technology which began to emerge in the 1950s with the idea of manipulating small volumes of fluids to allow controlled mixing and reaction, and has rapidly evolved into devices which miniaturise laboratory techniques with high sensitivity and specificity. Two main categories of device are available for the maintenance of cells and tissue, including droplet-based and continuous-flow, with and without the integration of analytical modules such as polymerase chain reaction and flow cytometry (see Tanweer et al.14 for a review of microfluidic devices in head and neck cancer). The current review focusses on continuous flow devices for the maintenance and interrogation of human tissue ex vivo, with the aim of preserving tissue in its 3D in vivo-like structure, retaining complex cell-to-cell and cell-to-matrix interactions.
Tissue-based microfluidics
Microfluidic tissue culture mimics the systems and pressures of the human body with continuous perfusion, allowing delivery of nutrients to multiple pieces of the same tissue in parallel, with the simultaneous removal of waste (Figure 1; page 00)15-16. The use of microfluidics to study human tissue offers an innovative method for studying the in vivo environment compared with cell lines – which are artificially immortalised for long-term culture and lack the cellular interactions – and animal models, which may not physiologically or genetically represent true tumour or human physiology.
The culture of human tissue in a chip and more importantly human tumour tissue in a chip allows for the correct micro-environment to be maintained ex vivo and for stromal-epithelial interactions to be studied14. The maintenance of tissue in this manner allows the effect of various drug combinations, irradiation, and chemical reactions to be monitored and assessed with the potential of identifying optimal treatment strategies for the individual in a timely manner prior to first administration to the patient. For example van Midwoud et al.17 described how microfluidics was used in combination with precision-cut liver slices as an improved biomimetic system,17 incorporating a micro-chamber for slice “perfusion” under continuous flow to perform drug metabolism studies. Alternatively, Sylvester et al.16 monitored the ability of chemotherapeutic drug combinations to kill head and neck tumour tissue (HNSCC) biopsies.
Since the first evidence of microfluidic culture of rat brain tissue slices in 200318, various devices have been designed and utilised for the culture of both animal and human tissue under ex vivo conditions, the most recent of which (since 2013) are summarised in Table 1 (page 00). These studies show the diverse range of uses that microfluidic tissue culture can be applied to, from the analysis of cell migration in the retina19 through to the commercialisation of the artery-on-a-chip model, to assess calcium dynamics and vascular tone20-21. Studies such as that by Chang et al.22 demonstrate the intricate delivery of parallel drugs simultaneously or sequentially to the same piece of mouse brain tissue and are able to overcome the problems of tumour heterogeneity between patients and between tumours of different origin; a further benefit is the ability to establish multiple biopsies to assess tumour heterogeneity. Research at the University of Hull has focussed on the use of a simple, continuous flow, glass chip design (Figure 2; page 00) for the maintenance and interrogation of a selection of human tissues, described below.
Head and neck cancer on a chip
Extensive work has been carried out using HNSCC maintained in microfluidic devices in which the effects of chemotherapeutic drugs, alone and in combination, and external beam radiation have been investigated in terms of cell death and proliferation16,25,28. Using the glass microfluidic device (Figure 2), head and neck tumour tissue weighing approximately 10mg with a volume of 8mm3 was maintained for nine days to demonstrate the synergistic cytotoxic effects of chemotherapeutic drug combinations28. Further experiments extended the tissue incubation period out to 15 days allowing the assessment of tissue response to irradiation. It was demonstrated that apoptotic response to irradiation could be detected in the tissue following as little as 2Gy, that the effect was dose dependant and that a fractionated course of 5 x 2Gy, similar to that received by patients, demonstrated a significantly greater apoptotic response compared with the single 2Gy dose25. The combination of these studies with on-chip analyses, such as those developed by Woods et al.29 and others30-31, not only provides a unique platform for the development of assays to predict response of any solid tumour to various treatments prior to commencing treatment, but also allows the investigation of tumour biology in a pseudo in vivo environment without the need for animal experimentation.
Cardiac tissue on a chip
A similar device was developed to maintain heart tissue samples (Figure 2) taken from either a human or rat with the addition of electrical stimulation to emulate the beating of heart tissue in vivo. The viability of the cardiac tissue was determined by biochemical measurement of the release of lactate dehyrogenase (LDH) and hydrogen peroxide as markers of tissue damage. This device also allowed primary investigations into electrochemical monitoring of reactive oxygen species and lactate during perfusion of the tissue to address clinically relevant issues such as ischemia reperfusion32.
Liver tissue on a chip
Work by Hattersley et al.33 repeatedly demonstrated the ability of the Hull microfluidic device to maintain viable, functional liver tissue for up to 72 hours, measured using LDH and water soluble tetrazolium-1 release, histology, as well as albumin and urea production. This work provided a platform to study toxicity whilst the liver was perfused with ethanol33. The ability to measure changes in production of a normal tissue product in the effluent over time, with varying concentrations of ethanol assault, show the power of the approach for real-time monitoring.
Pitfalls and limitations of microfluidic culture
Microfluidics has yet to make the impact on science it deserves, as the technology requires a range of subsystems and components with the integration of complete systems. As G.M Whitesides, a founding father of this technology stated, “the field of microfluidics is in early adolescence, and still lacks both these essential requirements, in addition to the integration of components into systems that can be used by non-experts. As a field, it is a combination of unlimited promise, pimples and incomplete commitment. This is a very exciting time for the field, but we still do not know exactly what it will be when it grows up” 34. This comment was made in 2006 but remains true for clinical use in 2016 as the combination of scientific disciplines has not yet truly worked together to fulfil the technology’s potential.
To date, much of the tissue-on-chip research has focussed on demonstrating the ability of a microfluidic device to measure a particular analyte in the effluent or tissue, following some form of treatment. These studies tend to be led by either biology or chemistry researchers approaching a question from their discipline and many of these studies have shown it is possible to study individual parameters. The ability of these parameters to become integrated in a way to address a bigger question such as delivering personalised medicine, or offering a robust methodology for screening off-target organ drug effects, has not yet been demonstrated. One of the reasons for this is the need for long-term investment and the input of multiple scientific disciplines, for example, clinicians, engineers and modellers in addition to chemists and biologists as the coupling of tissue to ‘downstream’ analysis modules requires compromises in microfluidic chip design, tissue maintenance and analyte detection. However, the goals of improved treatment at reduced cost whilst using fewer animals provides a strong impetus for the technology to deliver.
Impact towards reducing animal models
The majority of current clinical research methodology uses animal models that are induced into the diseased state and treated with test substances at some time in their lives. Despite many known limitations, these animal models are commonly used, and for drug testing are often explicitly required. An aim of tissue-on-a-chip research is to reduce the number of animals used for the study of cancer and its treatment, firstly because increasingly data suggest the animal models do not adequately represent the human disease but also because the morality for the use of vast numbers of animals in research is continually being questioned. According to UK Home Office statistics there has been an increase year on year in the number of animals used for research since 1995, and in 2013 the number of animals used in cancer research was 500,769 (UK’s latest statistics of Scientific Procedures on Living Animals), a 13% increase from the previous year35. The adoption of microfluidic technology to maintain patient biopsies could offer a viable replacement option for many animal models, being not only cost effective but simple to mass produce and operate, allowing for better science in the future, using human tissue to represent human disease.
Conclusion
Microfluidic tissue culture is well on the way to becoming a reputable substitute for animal models in the study of human disease, but investment and wide-spread adoption are required to deliver the new platforms and concomitant health benefits.
References
- Siegel R, Ma J, Zou Z, Jemal A. Cancer statistics, 2014. CA: A Cancer Journal for Clinicians. 2014;64(1):9-29
- Hay M, Thomas DW, Craighead JL, Economides C, Rosenthal J. Clinical development success rates for investigational drugs. Nat Biotech. 2014;32(1):40-51
- Maker AV, Ito H, Mo Q, Weisenberg E, Qin L-X, Turcotte S, et al. Genetic evidence that intratumoral T-cell proliferation and activation is associated with recurrence & survival in patients with resected colorectal liver metastases. Cancer Immunol Res. 2015:3(4):380-8
- Tobin NP, Harrell JC, Lövrot J, Egyhazi Brage S, Frostvik Stolt M, Carlsson L, et al. Molecular subtype and tumor characteristics of breast cancer metastases as assessed by gene expression significantly influence patient post-relapse survival. Ann Oncol. 2015;26(1):81-8
- Restifo NP, Smyth MJ, Snyder A. Acquired resistance to immunotherapy and future challenges. Nat Rev Cancer. 2016;16(2):121-6
- Pienta KJ, Abate-Shen C, Agus DB, Attar RM, Chung LWK, Greenberg NM, et al. The current state of preclinical prostate cancer animal models. The Prostate. 2008;68(6):629-39
- Block KI, Gyllenhaal C, Lowe L, Amedei A, Amin ARMR, Amin A, et al. Designing a broad-spectrum integrative approach for cancer prevention and treatment. Semin Cancer Biol. 2015;35, Supplement:S276-S304
- Kim HJ, Huh D, Hamilton G, Ingber DE. Human gut-on-a-chip inhabited by microbial flora that experiences intestinal peristalsis-like motions and flow. Lab Chip. 2012;12(12):2165-74
- Kim JB. Three-dimensional tissue culture models in cancer biology. Semin Cancer Biol. 2005;15(5):365-77
- Williamson A, Singh S, Fernekorn U, Schober A. The future of the patient-specific Body-on-a-chip. Lab Chip. 2013;13(18):3471-80
- Leonard F, Godin B. 3D In Vitro Model for Breast Cancer Research Using Magnetic Levitation and Bioprinting Method. In: Cao J, editor. Breast Cancer: Methods and Protocols. New York, NY: Springer New York; 2016. p. 239-51
- Stebbing J, Paz K, Schwartz GK, Wexler LH, Maki R, Pollock RE, et al. Patient-derived xenografts for individualized care in advanced sarcoma. Cancer. 2014;120(13):2006-15
- Malaney P, Nicosia SV, Davé V. One mouse, one patient paradigm: New avatars of personalized cancer therapy. Cancer Lett. 2014;344(1):1-12
- Tanweer F, Louise Green V, David Stafford N, Greenman J. Application of microfluidic systems in management of head and neck squamous cell carcinoma. Head Neck. 2013;35(5):756-63
- Hattersley SM, Greenman J, Haswell SJ. The application of microfluidic devices for viral diagnosis in developing countries. Methods Mol Biol 2013;949:285-303
- Sylvester D HS, Stafford ND, Haswell SJ, Greenman J. Development of microfluidic-based analytical methodology for studying the effects of chemotherapy agents on cancer tissue Current Analytical Chemistry. 2013;9 (1) 2-8
- van Midwoud PM, Groothuis GMM, Merema MT, Verpoorte E. Microfluidic biochip for the perifusion of precision-cut rat liver slices for metabolism and toxicology studies. Biotechnol Bioeng. 2010;105(1):184-94
- Passeraub PA AA, V TN. Design, microfabrication and characterization of a microfluidic chamber for the perfusion of brain tissue slices. Biomed Microdevices. 2003;5(147-155)
- Dodson KH, Echevarria FD, Li D, Sappington RM, Edd JF. Retina-on-a-chip: a microfluidic platform for point access signaling studies. Biomed Microdevices. 2015;17(6):1-10
- Yasotharan S, Pinto S, Sled JG, Bolz S-S, Gunther A. Artery-on-a-chip platform for automated, multimodal assessment of cerebral blood vessel structure and function. Lab Chip. 2015;15(12):2660-9
- Gunther A, Yasotharan S, Vagaon A, Lochovsky C, Pinto S, Yang J, et al. A microfluidic platform for probing small artery structure and function. Lab Chip. 2010;10(18):2341-9
- Chang TC, Mikheev AM, Huynh W, Monnat RJ, Rostomily RC, Folch A. Parallel microfluidic chemosensitivity testing on individual slice cultures. Lab Chip. 2014;14(23):4540-51
- Astolfi M, Peant B, Lateef MA, Rousset N, Kendall-Dupont J, Carmona E, et al. Micro-dissected tumor tissues on chip: an ex vivo method for drug testing and personalized therapy. Lab Chip. 2016;16(2):312-25
- Zambon A, Zoso A, Gagliano O, Magrofuoco E, Fadini GP, Avogaro A, et al. High Temporal Resolution Detection of Patient-Specific Glucose Uptake from Human ex Vivo Adipose Tissue On-Chip. Anal Chem. 2015;87(13):6535-43
- Carr SD GV, Stafford ND, Greenman J. Analysis of radiation-induced cell death in head and neck squamous cell carcinoma and rat liver maintained in microfluidic devices Otolaryngol Head Neck Surg. 2014;150(1):73-80
- Silva PN, Green BJ, Altamentova SM, Rocheleau JV. A microfluidic device designed to induce media flow throughout pancreatic islets while limiting shear-induced damage. Lab Chip. 2013;13(22):4374-84
- Atac B, Wagner I, Horland R, Lauster R, Marx U, Tonevitsky AG, et al. Skin and hair on-a-chip: in vitro skin models versus ex vivo tissue maintenance with dynamic perfusion. Lab Chip. 2013;13(18):3555-61
- Hattersley S, Sylvester D, Dyer C, Stafford N, Haswell S, Greenman J. A microfluidic system for testing the responses of head and neck squamous cell carcinoma tissue biopsies to treatment with chemotherapy drugs. Ann Biomed Eng. 2012;40(6):1277-88
- Woods J, Docker PT, Dyer CE, Haswell SJ, Greenman J. On-chip integrated labelling, transport and detection of tumour cells. Electrophoresis. 2011;32(22):3188-95
- Erickson D, Li D. Integrated microfluidic devices. Analytica Chimica Acta. 2004;507(1):11-26
- Liberale C, Cojoc G, Bragheri F, Minzioni P, Perozziello G, La Rocca R, et al. Integrated microfluidic device for single-cell trapping and spectroscopy. Scientific Reports. 2013;3:1258
- Cheah LT, Dou YH, Seymour AML, Dyer CE, Haswell SJ, Wadhawan JD, et al. Microfluidic perfusion system for maintaining viable heart tissue with real-time electrochemical monitoring of reactive oxygen species. Lab Chip. 2010;10(20):2720-6
- Hattersley SM, Greenman J, Haswell SJ. Study of ethanol induced toxicity in liver explants using microfluidic devices. Biomed Microdevices. 2011;13(6):1005-14
- Whitesides GM. The origins and the future of microfluidics. Nature. 2006;442(7101):368-73
- https://gov.uk/government/collections/statistics-of-scientific-procedures-on-living-animals
Biographies
Dr Amy Dawson is a post-doctoral researcher using microfluidic technology to develop a dual-flow device for the maintenance of human intestinal tissue in a known orientation. The dual-flow maintains the two surfaces of the intestine separately with the aim focused on the treatment of inflammatory bowel disease and establishing the interaction of the tissue with the commensal bacteria present in the gut. This is part of a collaborative study with Scarborough General Hospital and the Østfold Hospital Trust, Norway. Dr Dawson studied a BSc, MSc in biomedical science and a PhD in chemistry at the University of Hull.
Dr Victoria Green coordinates the head and neck/thyroid cancer research group which uses microfluidic technology to maintain and interrogate biopsies of head and neck/thyroid tumours. Tissue response is investigated with a view towards prediction of treatment outcome. Other research involves the role of the host’s immune system in cancer, looking at the balance of immune cells, with a focus on T regulatory (Treg) cells and cytotoxic T cells. Dr Green achieved a first class (Hons) degree in Biochemistry at the University of York before completing her PhD at the University of Hull where she is now a post-doctoral researcher.
Ruth Bower is currently researching head and neck cancer chemo-radiotherapy regimens on a microfluidic platform. The tissue is maintained and the response to treatment assault is monitored using a variety of whole tissue and cellular analytical techniques. Ultimately this technology could lead to treatment selection on a patient by patient basis. Ruth Bower achieved a first class (Hons) degree in Biological Sciences at Lancaster University before moving the University of Hull where she is now a PhD candidate.
Prof John Greenman is a tumour immunologist by background but for the past decade has worked collaboratively with chemists, clinicians and engineers to develop lab-on-a-chip technology for analysing tumour biopsies. This approach allows the response to various interventions to be determined on an individual patient basis. The majority of his research has focused on head and neck tumours, however the methodology has been applied to other tissues, including heart. John was awarded Heart Research UK’s Outstanding Researcher of the year in 2012. Prior to joining the University of Hull in 1995, where he is Head of the School of Biological Biomedical & Environmental Sciences, he worked at Oxford University.
Related topics
Microfluidic Technology, Personalised Medicine
Related organisations
Hull University
Related people
Amy Dawson, John Greenman, Ruth Bower, Victoria Green
That’s great, please do get rid of the translational complications that animal models bring!