A bright future for molecular probes
Posted: 24 September 2015 | Rudi Marquez (University of Glasgow), Zuzana Flachbartova (University of Veterinary Medicine and Pharmacy in Kosice) | No comments yet
The history of molecular probes can be traced back to 1871 with the synthesis of fluorescein by Adolf von Baeyer1. Through the simple combination of phthalic anhydride and resorcinol in the presence of zinc chloride, the dawn of a completely new era in chemistry and biology began…
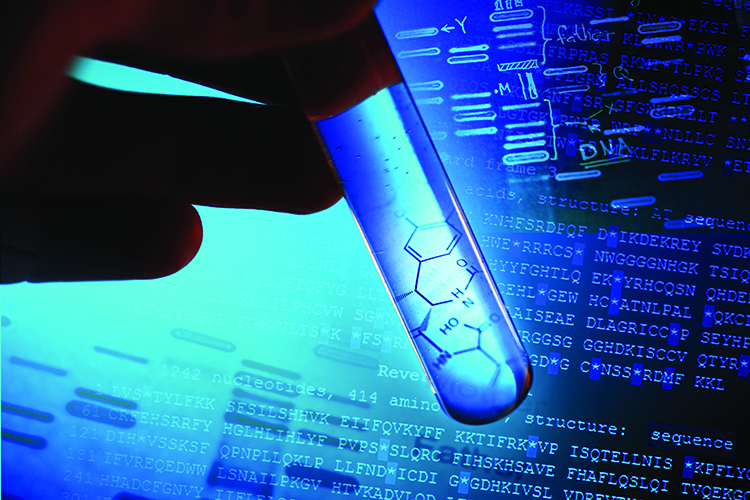
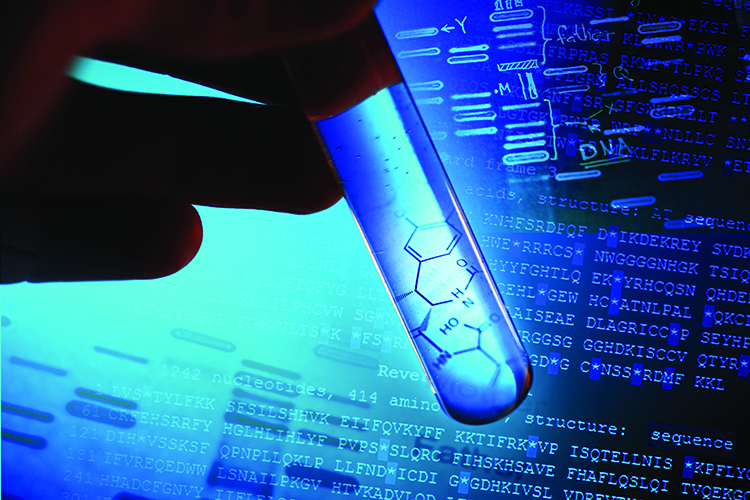
Indeed within a few years other xanthene dyes such as rhodamine b had been reported and patented2. Not surprisingly, over time, xanthene-derived dyes found widespread use in a number of areas covering a wide range of medical, environmental and industrial applications.
A few years after fluorescein, the cyanine dyes (Cy dyes) were first synthesised. Structurally very different from the xanthene dyes, the cyanine dyes were initially designed for the photographic industry. They are based around a triene or a pentaene core and provide access to a wider range of absorption and emission wavelengths combined with lower toxicity issues and improved solubility in aqueous systems3. As such, cyanine dyes have found widespread use in the labelling of nucleotides, proteins and peptides. Unfortunately, the cyanine dyes have been plagued with stability issues though, as well as unpredicted wavelength shifts upon substrate conjugation.
The solubility and stability issues that were encountered with the xanthene- and cyanine-based dyes provoked the development of the Alexa Fluor family of fluorescent dyes. The Alexa Fluor dyes are derived from the chemical modification of the xanthene, coumarin and cyanine frameworks. As expected, these dyes provide a similar range of excitation and emission wavelengths as the parent compounds. However, they display enhanced brightness and photostability, which combined with their water solubility and pH stability has made them key tools in current biological research4.
The chemical structures of the first wave of dyes are depicted in Figure 1.
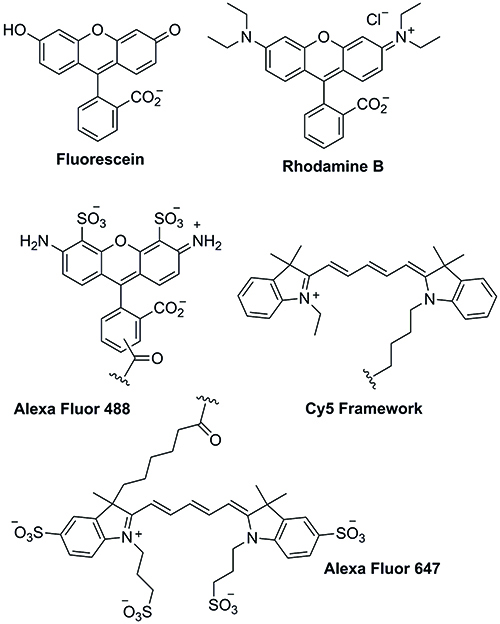
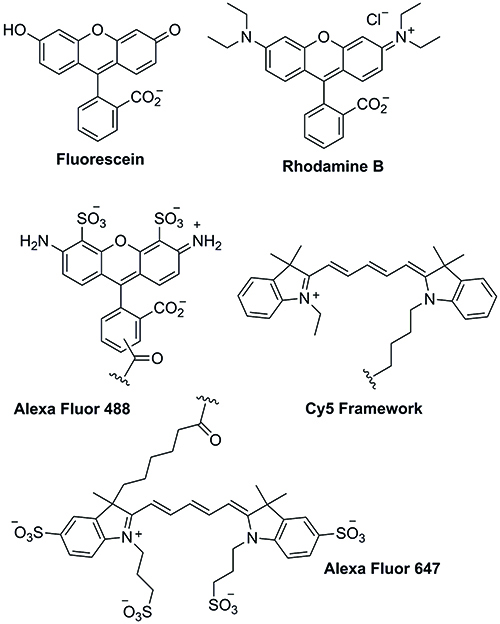
Figure 1: Xanthene- and cyanine-based dyes.
A new class of dyes
The BODIPY (4,4-difluoro-4-bora-3a,4a-diaza-s-indacene) dyes are relatively new arrivals in the field of fluorescent dyes. The first BODIPY dyes synthesised (by Kreuzer) were the 1,3,5,7-tetramethyl-2-acetyl BODIPY and 1,3,5,7-tetramethyl-2,6-diacetyl BODIPY using formylating conditions on 2,4-dimethylpyrrole5.
The BODIPYs are quickly becoming some the most useful fluorescent tags due to their inherently user-friendly properties, including large molar absorption coefficient, tunable fluorescence and high quantum yields (60-90% on average), which is responsible for their brightness. The BODIPYs also exhibit excellent photostability, and a small Stokes shift between their absorption and emission wavelengths which are located in the visible spectrum and are not affected by the choice of solvent used. The BODIPY dyes are stable to changes in pH, are robust in varying physiological conditions, and most importantly are cell permeable and non-toxic. Thus, it is not surprising that a large number of BODIPY analogues have been generated and a set of them is commercially available6.
The BODIPYs are also attractive due to their relatively straightforward synthesis. There are a number of strategies available for the synthesis of both symmetrical and unsymmetrical BODIPY dyes. Symmetrical BODIPY dyes tend to be synthesised by incorporation of the meso substituent already in place through the coupling of two equivalents of the desired pyrrole and the required acid chloride. The dipyrromethene product resulting from this coupling is then treated in situ with BF3.OEt2 to yield the BODIPY core (Figure 2).
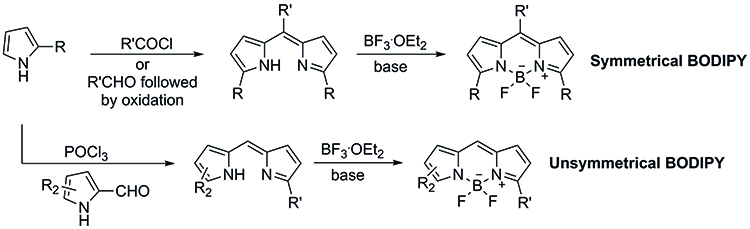
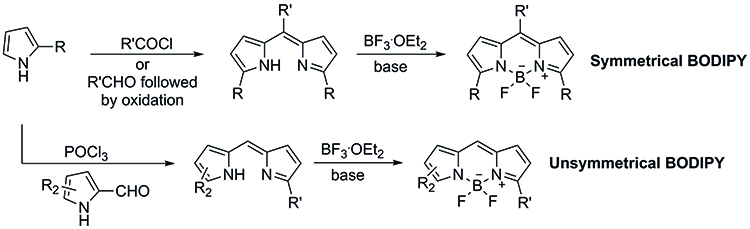
Figure 2: The synthesis of symmetrical and unsymmetrical BODIPY dyes.
An alternative approach to the synthesis of symmetrical BODIPY dyes involves the use of the desired pyrrole with a required aldehyde which will become the meso substituent, known as the Lindsey method. This approach requires the use of an oxidation step to generate the dipyrromethene intermediate which can then be converted into the corresponding BODIPY.
Unsymmetrical BODIPY dyes require the formylation of one of the pyrrole starting units before the condensation with a second pyrrole in the presence of POCl3. Treatment of the resulting dipyrromethene intermediate with BF3.OEt2 yields the desired unsymmetrical fluorescent dye.
Thus, it is not surprising that the BODIPY dyes have quickly gained popularity in the last few years, and the interest of the chemical and biological communities on these compounds is ever expanding with over 1700 papers and patents being published in the last two years alone.
The real power of the BODIPY dyes, however, resides in their potential ability to undergo a wavelength shift upon selective chemical or biological functionalisation of the diaza-indacene core. One of the most studied modifications of the BODIPY core requires the generation of Biellman BODIPY (8-methylthio-BODIPY) analogues. The synthesis of 8-thioether BODIPY analogues begins with the generation of the thioketone intermediate from the corresponding pyrroles. Treatment of thioketone with methyl iodide then affords the dipyrrolomethylene unit, which can then be converted into the desired 8-thioether-BODIPY derivatives7,8.
Indeed, reaction of different amines with unsubstituted 8-thioether BODIPY results in the generation of 8-amino-BODIPY dyes. The electron-donating amino groups cause a significant blue-shift of the absorption and emission spectral bands accompanied by a large Stokes shift. Alcohols and phenols have also been successfully used for the functionalisation of 8-methylthio-BODIPY via SNAR processes. Unfortunately, the blue-shift associated with the introduction of electron rich substituents at the meso position of the BODIPY core has significantly reduced the impact of 8-thioether-BODIPY dyes in both chemical and biological applications.
Chlorinated BODIPY dyes on the other hand provide some of the most exciting recent developments in the areas of fluorescent molecular probes and imaging. 3,5-Dichloro-BODIPY dyes can be easily synthesised through the NCS chlorination of the Lindsey bis-pyrrole intermediate. Oxidation, followed by treatment with BF3.OEt2 then completes the synthesis of the 3,5-dichloro-BODIPY unit9.
The potential versatility of the 3,5-dichloro-BODIPY dyes was first demonstrated by the pioneering work of Dehaen and Boens, who explored the use of direct functionalisation of the fluorescent dye through a selective nucleophilic addition10,11. Dehaen showed the potential to use oxygen, nitrogen, sulphur and carbon centered nucleophiles, while developing experimental conditions for the mono- or disubstitution of the dichloro-BODIPY dye (Figure 3).
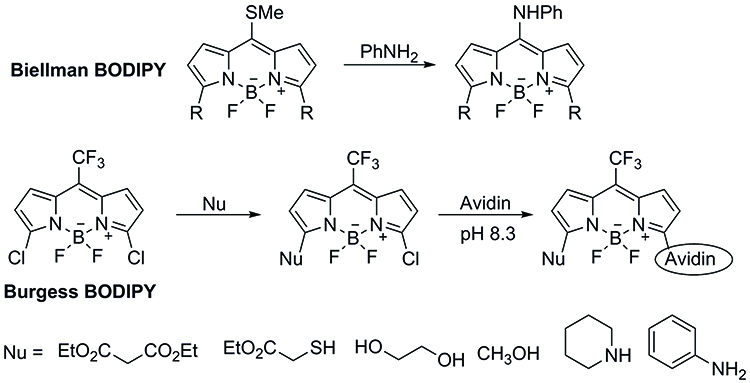
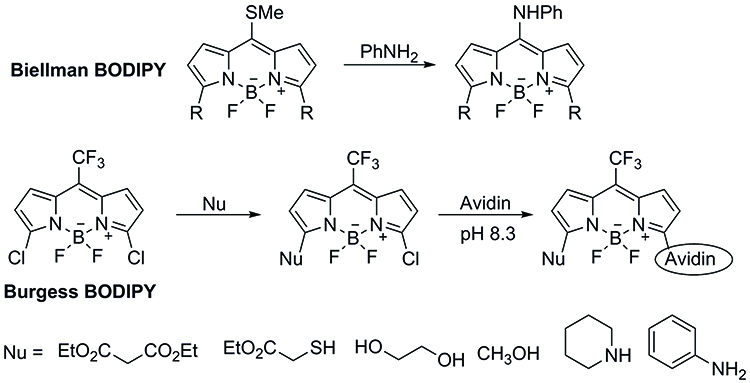
Figure 3: The potential versatility of the 3,5-dichloro-BODIPY dyes was first demonstrated by the pioneering work of Dehaen and Boens, who explored the use of direct functionalization of the fluorescent dye through a selective nucleophilic addition.
Using the experimental conditions developed in their laboratories, Dehaen and Boens were able to generate asymmetric BODIPY dyes not easily generated through other approaches. As expected, introduction of substituents at the 3- and 5- positions of the BODIPY core results in a shift in the absorption and emission spectra as well as affecting the fluorescence quantum yield. It is worth pointing out that the shifts in emission and absorption spectra of the 3- and 5- substituted BODIPY dyes are smaller than those observed through meso substitution.
Building upon the key results of Dehaen and Boens, Burgess developed a 3,5-dichloro-BODIPY dye incorporating a trifluoromethyl unit at the meso position of the BODIPY framework. The trifluoromethyl unit at the meso position was expected to increase the electrophilicity of the BODIPY dye, making it a more likely candidate for direct protein labelling. Furthermore, the trifluoromethyl unit would also provide a very useful NMR marker12.
Burgess’ synthesis of the trifluoromethyl probe began with the condensation of pyrrole with trifluoromethyl acetate. Di-chlorination of the bis-pyrrole residue followed by DDQ oxidation and treatment with BF3.OEt2 completed the synthesis of the Burgess trifluoromethyl-BODIPY dye.
As expected, the trifluoromethyl-BODIPY dye reacted with different sulphur and nitrogen bearing nucleophiles in good yields to afford the mono-chlorinated substituted BODIPY dyes. Excitingly, the monochlorinated BODIPY dye was then able to undergo coupling with avidin, further demonstrating the potential of this methodology.
Expanding potential of BIODIPY dyes
Recently, Feng and Yang have further expanded the use of the chloro-BODIPY dyes to develop BODIPY-based fluorometric sensors for the simultaneous determination of cysteine, homocysteine and glutathione. The quantitative determination of cysteine-, homocysteine- and glutathione-containing biological units has long been a target due to the significant role these units play within biological systems and for their potential to be used as disease biomarkers in a number human ailments. The simultaneous detection of these three units presents a significant challenge due to their structural similarity and large difference in physiological concentrations13,14.
Feng’s simultaneous detection approach is based on the differential reactivity of the 3-chloro-BODIPY dye towards glutathione, cysteine and homocysteine. In Feng’s system, glutathione reacts with the 3-chloro-BODIPY dye to generate the fluorescent glutathione BODIPY derivative A, which then undergoes no further reaction. Cysteine and homocysteine, on the other hand, couple with the 3-chloro-BODIPY dye to afford the thioether intermediates B and C. Thioether B can then undergo a kinetically favoured intramolecular rearrangement to produce the newly fluorescent amino-BODIPY derivative D, while thioether C remains unchanged. The differential reactivity between the two thioether units is dictated by the difference in the transition states during the potential sulphur to nitrogen transfers. Thus after a given period of time, any mixture of glutathione, cysteine and homocysteine should afford complexes A, C and D respectively in the presence of excess of 3-chloro-BODIPY (Figure 4).
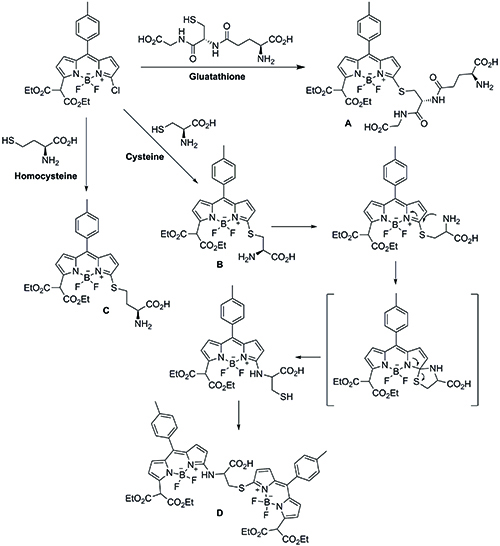
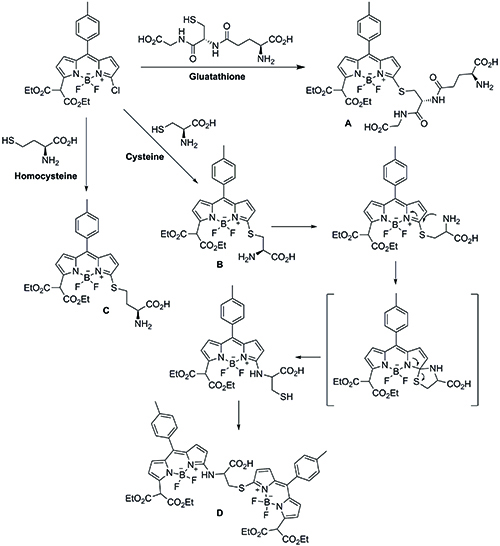
Figure 4: A schematic of Feng’s simultaneous detection approach.
Rewardingly, treatment of human serum samples with excess 3-chloro-BODIPY generated the three expected addition substrates A, C, and D after one hour. Significantly, the large difference in polarities between the three substrates allowed their separation by thin layer chromatography, avoiding the need for high-performance liquid chromatography separation. Furthermore, the presence of the fluorescent BODIPY tag also allowed for their successful quantification using molecular imaging techniques.
Using this novel approach, Feng was able to successfully separate and determine the glutathione, cysteine and homocysteine levels within human serum without the need for HPLC separation. The concentration of the three biological units matches those obtained through traditional HPLC methods reported in the literature.
Conclusion
There are a large number of fluorescent probes available commercially for the tagging and labelling of compounds and proteins of biological interest using a variety of chemical ligation techniques. While it could be argued that in simple cases, the AlexaFluor dyes are the reagents of choice due to their brightness and stability, recent results showcase the power and potential of the BODIPY dyes in biological chemistry. BODIPY- based dyes provide excellent physicochemical and biological properties combined with the unparalleled potential to be modified and applied to different sensing and visualisation applications. The demonstrated ability of the BODIPY dyes to change their emission and absorption spectra upon binding make them the reagents of choice for more demanding applications. The current interest of the chemical and biological communities on the use and application of BODIPY dyes points to a bright future for the BODIPY dyes in biological, medicinal and materials applications.
Biographies
References
- Von Bayer, A. Chem. Ber. 1871, 5, 255
- Beija, M.; Afonso, C. A. M.; Martinho, J. M. G. Chem. Soc. Rev. 2009, 38, 2410
- Berlier, J. E.; Rothe, A.; Buller, G.; Bradford, J.; Gray, D. R.; Filanoski, B. J.; Telford, W. G.; Yue, S.; Liu, J.; Cheung, C -Y.; Chang, W.; Hirsh, J. D.; Beechem, J. M.; Haugland, R. P.; Haugland, R. P. J. Histochem. Cytochem. 2003, 51, 1699
- Panchuk-Voloshina, N.; Haugland, R. P.; Bishop-Stewart, J.; Bhalgat, M. K.; Millard, P. J.; Mao, F. Leung, W-Y.; Haugland, R. P. J. Histochem. Cytochem. 1999, 47, 1179
- Treibs, A.; Kreuzer, F-H. Liebigs Ann. Chem. 1968, 718, 208
- Ulrich, G.; Ziessel, R.; Harriman, A. Angew. Chem. Int. Ed. 2008, 47, 1184
- Goud, T, V.; Tutar, A.; Biellman, J -F. Tetrahedron, 2006, 62, 5084
- Esnal, I.; Urias-Benavides, A.; Gomez-Duran, C. F. A.; Osorio-Martinez, C. A.; Garcia-Moreno, I.; Costela, A.; Banuelos, J.; Epelde, N.; Arbeola, I. L.; Hu, R.; Tang, B. Z.; Pena-Cabrera, E. Chem. Asian J. 2013, 8, 2691
- Lakshmi, V.; Rao, M. R.; Ravikanth, M. Org. Biomol. Chem. 2015, 13, 2501
- Rohand, T.; Baruah, M.; Qin, W.; Boens, N.; Dehaen, W. Chem. Commun. 2006, 266
- Baruah, M.; Qin, W.; Vallee, R. A. L.; Beljonne, D.; Rohand, T.; Dehaen, W.; Boens, N. Org. Lett. 2005, 7, 4377
- Li, L.; Nguyen, B.; Burgess, K. Bioorg. Med. Chem. Lett. 2008, 18, 3112
- Jia, M –Y.; Niu, L –Y.; Zhang, Y.; Yang, Q –Z.; Tung, C –H.; Guan, Y –F.; Feng, L. ACS Appl. Mater. Interfaces 2015, 7, 5907
- Wang, F.; Zhou, L.; Zhao, C.; Wang, R.; Fei, Q.; Luo, S.; Guo, Z.; Tian, H.; Zhu, W-H. Chem. Sci. 2015, 6, 2584
Related topics
Imaging, Molecular Biology