A new era of genetic and mechanistic molecular neuroscience
Posted: 17 May 2024 | Dr Tarek Deeb (AstraZeneca), Dr Zhong Zhong (Ovid) | No comments yet
Neuroscientists are increasingly viewing disorders of the brain through the lens of the underlying molecular mechanisms as sometimes illuminated by genetic variants, rather than classifying disorders based solely on the clinical symptoms.
The next step in the evolution of antiseizure medication will likely come from studying these molecular determinants of the various epilepsy disorders, and using a precision medicine approach to develop new drugs that dampen neuronal hyperexcitability without typical side effects.
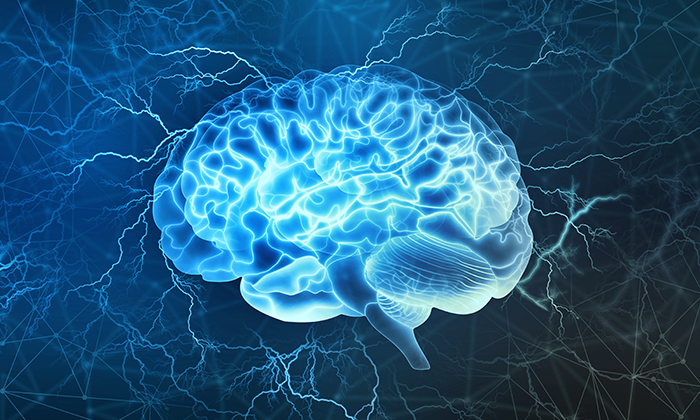
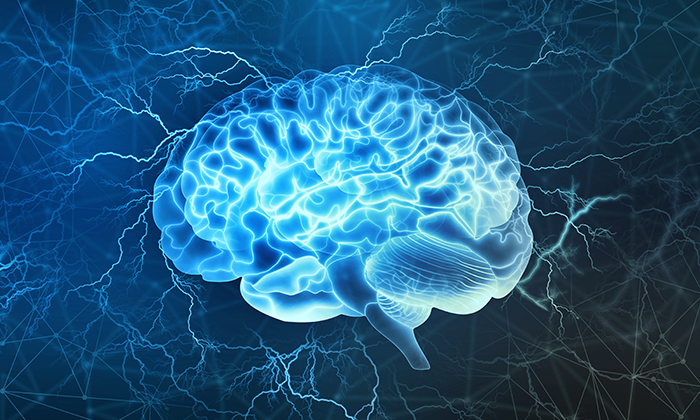
The field of neuroscience is increasingly shifting away from a purely macroscopic view of brain disorders and towards a far more integrated approach involving detailed analyses of the molecular underpinnings of disease.
Importantly and excitedly, this change in perspective suggests that what we determine as boundaries between different neurological disorders may be superficial. A closer inspection reveals that at the etiology and the underlying biology of seemingly distinct neurological conditions have some similarities in genetic and mechanistic drivers.
Reclassifying complex neurological disorders based on new genomic, transcriptomic, and proteomic datasets is leading drug discovery to new targets and towards the regulation of critical pathways underlying the disease biology itself. Alongside a technological revolution fueled by machine learning, artificial intelligence, and various breakthroughs in imaging and biomarkers – small molecule neuropharmacology is poised for major advances that will impact millions of lives.
Epilepsy is an example of ongoing therapeutic innovation that serves to highlight the need for new approaches
For over 100 years the therapeutic landscape of antiseizure medications (ASM) has evolved extensively. The ASMs treatment armamentarium offers a wide range of therapies targeting more than a half dozen mechanisms of action (MOAs): sodium or calcium channel blockers, GABAA receptor modulators, GABA reuptake inhibitors, GABA transaminase inhibitors, ionotropic glutamate receptor blockers, inhibitors of neurotransmitter release, and neuronal potassium channel openers, among others.
Undoubtedly, epilepsy is a success story in terms of innovations delivered to patients. Yet, there is no epilepsy cure per se. Further, about 3 out of 10 people do not achieve seizure control, and even when effective ASMs tend to alter neurotransmission in a way that often generates undesirable side effects.1
Understanding epilepsy as a condition of hyperexcitability
All seizures share a common state of hyperexcitability at the level of individual neurons and synchronisation at the level of neuronal microcircuits and across brain regions.2 Thus, current ASMs tend to curb hyperexcitability. The next evolution in ASM discovery is examining the molecular underpinnings of seizures and developing drugs with novel MOAs to achieve control of neuronal hyperexcitability using a precision medicine approach.3-8
Most current therapies tend to tip the balance of neuronal excitation towards more inhibition, and in the process struggle to strike a balance of effective seizure control versus excessive neuronal inhibition leading to cognitive impairment, excessive tiredness, and somnolence. Thus, further attempts to directly tweak the excitatory/inhibitory balance will likely cause the same side effects unless new targets with novel MOAs are identified and clinically validated.
Targeting KCC2 as a mechanism to balance hyperexcitability
The major inhibitory neurotransmitter in the CNS is GABA, and its cognate receptor is the chloride-permeable GABAA receptor that mediates the actions of drugs such as benzodiazepines. Further studies have revealed the neuron-specific potassium-chloride cotransporter type 2 (KCC2) as a key regulator of ion homeostasis and GABAA function in neurons.9-11 Critically, KCC2 loss-of-function mutations in humans are associated with epilepsy, and studies on human brain tissue resected from patients with treatment-resistant epilepsy or brain tumours show downregulation of KCC2.12-16 This human data is particularly important given that genetic links to a drug’s MOA improve the probability of it having success in the clinic.17
KCC2 transports chloride ions from inside the neuron into the extracellular space, creating an inwardly directed gradient for the negatively charged chloride ions to flow back through the GABAA receptor whenever GABA is released in synapses. This allows GABAA receptors, and drugs that act on them, to perform their normal role as suppressors of neuronal excitability. But during a seizure, the chloride gradient collapses thus impairing the ability of GABAA receptors to inhibit the system, as well as reducing the therapeutic efficacy of GABAergic ASMs.
As an ion transporter, KCC2 exhibits self-limiting effects based on thermodynamic laws.18 As KCC2 extrudes more chloride and the cellular concentration falls toward the thermodynamic equilibrium point, its ion transporting activity automatically decreases. This acts as a potential built-in safety feature, and in this way, KCC2 activation should restore the excitatory-inhibitory balance without sedative side effects. In contrast, GABAA modulators, such as benzodiazepines, directly prolong inhibitory synaptic signals without similar built-in mechanisms that prevent overcompensation.
The KCC2 gene was discovered in 1997, and there have been several attempts to create indirect activators via transcriptional or post-translational mechanisms.19 However, these molecules have inherent tendencies to produce off-target biological effects and toxicities. The search for a direct activator of KCC2 first achieved success in the Stephen Moss laboratory at Tufts University. AstraZeneca, working with the Moss lab, conducted a high throughput screen of hundreds of thousands of compounds, and after the program was in-licensed by Ovid, the Moss lab continued to make important discoveries about its now lead candidate OV350. EEG studies in mice demonstrated that OV350 reached the brain and modulated brain activities consistent with activation of KCC2 transporters in neurons. With crosslinking studies, the Moss lab was able to demonstrate that OV350 directly binds to KCC2.20
In vivo proof-of-concept studies in animals have established that restoring KCC2 activity with OV350 leads to reduced seizure sensitivity and neuronal inflammation.20 In a preclinical model of status epilepticus (SE), OV350 in combination with diazepam terminated seizures, restored the efficacy of diazepam, and reduced associated neuronal loss following injury.20 Importantly, preclinical mechanistic studies have demonstrated OV350 is well-tolerated and did not induce sedation.20
Taking KCC2 and hyperexcitability beyond epilepsy
With the discovery of the first direct activator of KCC2, there is great hope this represents a new clinically validated MOA for ASMs, and one that fundamentally restores excitatory/inhibitory balance. But what is perhaps of greater consequence is how hyperexcitability, and thus KCC2, is an important underlying mechanism in many diseases beyond epilepsy, ranging from schizophrenia to neuropathic pain and Huntington’s disease.
Furthermore, today we are working with new tools and technologies, ranging from novel biomarkers, imaging, and radioligand PET tracers, to AI-powered reading of complex bioelectric phenomena (e.g., EEG) and digital biology (e.g., recording movement, attention span). This combination plus advanced molecular neuroscience promises a profound paradigm to shift in the treatment of disorders of the brain.
About the authors
Chief Scientific Officer, Ovid
As chief scientific officer, Dr Zhong leads all non-clinical scientific and research activities supporting the advancement of Ovid’s pipeline of potential first-in-class and best-in-class therapeutic candidates. Dr Zhong oversees Ovid’s scientific strategy, the Company’s research team, and academic collaborations with the goal of accelerating research underpinning the clinical development of the Company’s pipeline assets as well as its ROCK2 inhibition and KCC2 activator platforms.
Dr Zhong joined Ovid from Generation Bio where he served as Senior Vice President and oversaw DNA Science and R&D operations from 2019 – 2022. He was previously Vice President of Biology at Wave Life Sciences from 2016 – 2019, and led the discovery of oligotherapeutics for central nervous system, hepatic/metabolic, and muscle diseases. Previously, at Vertex Pharmaceuticals, he established a research site in Shanghai before moving to Boston in 2015 to oversee external research and innovation projects. From 2008 to 2013, as part of GSK’s leadership team for neuroscience R&D, Dr Zhong established both platform functions and a Regenerative Medicine Discovery Performance unit. He also led the GSK neuroinflammation R&D team, which delivered clinical candidates for several multiple sclerosis clinical studies.
Dr Zhong started his career at Johnson & Johnson, developing cell assay technologies as well as leading operations and research for the start-up Cell & Molecular Technologies through its acquisition and integration by Invitrogen (now Thermo Fisher Scientific).
Dr Zhong received his doctorate from The Rockefeller University where he discovered Stat3 and Stat4.
Former Biology leader of the KCC2 program at the AstraZeneca-Tufts University Lab
Dr Deeb brings forth over 20 years of dedicated expertise in GABAA receptor physiology and pharmacology, ion channels, and ion transporters. Recently, he assumed the role of Biology leader for the KCC2 drug discovery program at the AstraZeneca-Tufts University Lab for Basic and Translational Neuroscience Research. Under his stewardship, the program achieved a remarkable milestone by discovering the first-in-class direct activators of KCC2. With a decade-long immersion in KCC2-related research and a publication record comprising nearly 20 papers in the field, Dr Deeb’s expertise is widely recognised. His tenure at the AZ-Tufts Lab was marked by investigations of various neurological disorders, where his contributions helped advance multiple drug development programs in neuroscience. As a respected authority in ion channels and transporters, Dr Deeb remains deeply committed to pushing the boundaries of neuroscience research and drug discovery.
References:
- Löscher W, Potschka H, Sisodiya SM, Vezzani A. Drug Resistance in Epilepsy: Clinical Impact, Potential Mechanisms, and New Innovative Treatment Options. Pharmacol Rev. 2020 Jul;72(3):606-638. doi: 10.1124/pr.120.019539.
- Tóth K, et al. Hyperexcitability of the network contributes to synchronization processes in the human epileptic neocortex. J Physiol. 2018 Jan 15;596(2):317-342.
- Maillard PY, et al. Molecular and clinical descriptions of patients with GABAAreceptor gene variants (GABRA1, GABRB2, GABRB3, GABRG2): A cohort study, review of literature, and genotype-phenotype correlation. Epilepsia. 2022 Oct;63(10):2519-2533.
- Benke TA, et al. Clinical and therapeutic significance of genetic variation in the GRIN gene family encoding NMDARs. Neuropharmacology. 2021 Nov 1;199:108805.
- Beck VC, et al. Beyond Dravet Syndrome: Characterization of a Novel, More Severe SCN1A-Linked Epileptic Encephalopathy. Epilepsy Curr. 2019 Jul-Aug;19(4):266-268.
- Ogiwara I, et al. Nav1.1 localizes to axons of parvalbumin-positive inhibitory interneurons: a circuit basis for epileptic seizures in mice carrying an Scn1a gene mutation. J Neurosci. 2007 May 30;27(22):5903-14.
- Hawkins NA, et al. Screening of conventional anticonvulsants in a genetic mouse model of epilepsy. Ann Clin Transl Neurol. 2017 Apr 26;4(5):326-339.
- Genton P, Gelisse P, Thomas P, Dravet C. Do carbamazepine and phenytoin aggravate juvenile myoclonic epilepsy? Neurology. 2000 Oct 24;55(8):1106-9.
- Thompson SM, Gähwiler BH. Activity-dependent disinhibition. II. Effects of extracellular potassium, furosemide, and membrane potential on ECl- in hippocampal CA3 neurons. J Neurophysiol. 1989 Mar;61(3):512-23.
- Hübner CA, et al. Disruption of KCC2 reveals an essential role of K-Cl cotransport already in early synaptic inhibition. Neuron. 2001 May;30(2):515-24.
- Uvarov P, et al. A novel N-terminal isoform of the neuron-specific K-Cl cotransporter KCC2. J Biol Chem. 2007 Oct 19;282(42):30570-6.
- Stödberg T, et al. Mutations in SLC12A5 in epilepsy of infancy with migrating focal seizures. Nat Commun. 2015 Sep 3;6:8038.
- Saitsu H, et al. Impaired neuronal KCC2 function by biallelic SLC12A5 mutations in migrating focal seizures and severe developmental delay. Sci Rep. 2016 Jul 20;6:30072.
- Fukuda A, Watanabe M. Pathogenic potential of human SLC12A5 variants causing KCC2 dysfunction. Brain Res. 2019 May 1;1710:1-7.
- Pallud J, et al. Cortical GABAergic excitation contributes to epileptic activities around human glioma. Sci Transl Med. 2014 Jul 9;6(244):244ra89.
- Huberfeld G, et al. Perturbed chloride homeostasis and GABAergic signaling in human temporal lobe epilepsy. J Neurosci. 2007 Sep 12;27(37):9866-73.
- Minikel EV, Painter JL, Dong CC, Nelson MR. Refining the impact of genetic evidence on clinical success. Nature. 2024 Apr 17.
- Williams JR, Payne JA. Cation transport by the neuronal K+-Cl− cotransporter KCC2: thermodynamics and kinetics of alternate transport modes. Am J Physiol Cell Physiol. 2004;287:C919–C931.
- Payne JA. Functional characterization of the neuronal-specific K-Cl cotransporter: implications for [K+]o regulation. Am J Physiol. 1997 Nov;273(5):C1516-25.
- Data on file.
Related topics
Drug Delivery, Drug Development, Drug Discovery, Drug Discovery Processes, Neurosciences, Targets
Related organisations
Ovid
Related people
Dr Tarek Deeb (AstraZeneca), Dr Zhong Zhong (Ovid)