Differentiated therapies: Unlocking stem cell medicine with epigenetics
Posted: 12 December 2024 | Cameron Lee (Tune Therapeutics) | No comments yet
The human body has an incredible ability to repair itself, both from acute trauma and chronic damage that accumulates as we age. Here, Cameron Lee, Principal Scientist at Tune Therapeutics, reveals the potential of harnessing the body’s natural regenerative capabilities with epigenetic control of stem cells.
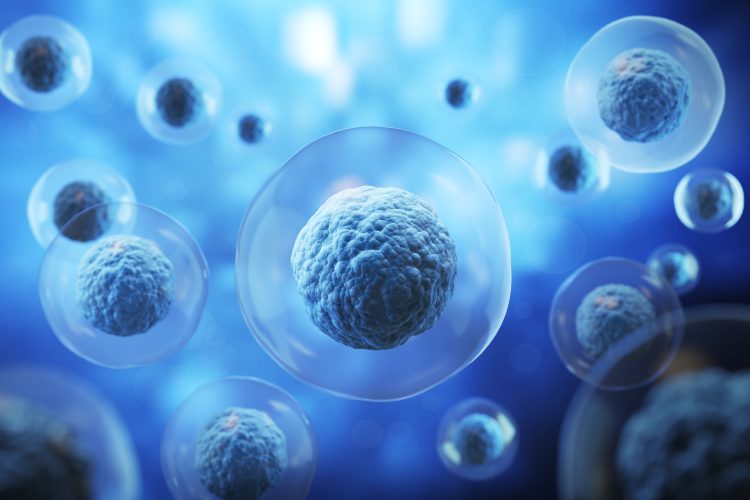
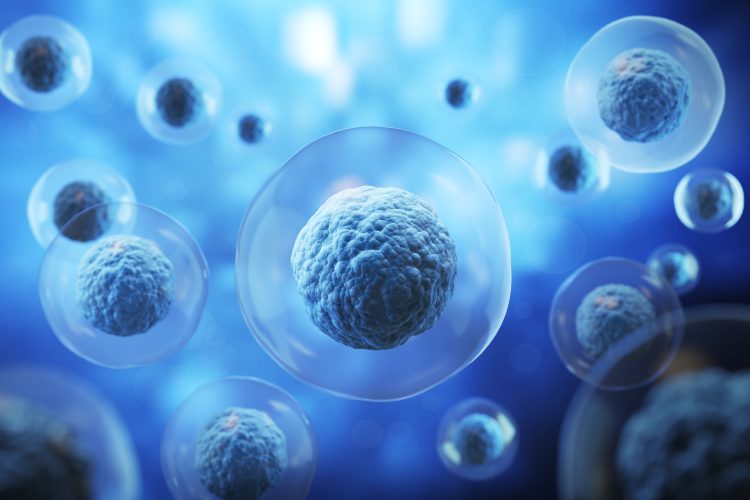
Stem cells often drive our bodies’ regeneration, with the capacity to differentiate into a myriad of different cell types that can mend or replace ageing or damaged tissue.
As result of this unique power, stem cells hold great therapeutic promise. But to realise their full potential in the space, we need to understand and commandeer the epigenetic processes that drive their differentiation. That final frontier is now within reach. Major scientific leaps have brought us closer than ever to harnessing the power of epigenetics to shape stem cell journeys for scalable therapeutic production.
The stem cells within: iPSCs
A variety of stem cells have been identified in different locations of the body and at different stages of human development. Some of these naturally occurring stem cells are already being deployed in clinical-stage programmes. ViaCyte and Vertex, for example, are currently studying the use of embryonic stem cells (ESCs) to treat type 1 diabetes with promising preliminary results.1 However, ESCs are far from ideal. Aside from the ethical questions they raise, ESCs are in limited supply and are generally recognised as foreign by the recipient patient’s immune system. This can trigger an immune response that causes the body to reject these therapeutic cells. As a result, patients receiving ESC-derived therapies must also take immunosuppressive drugs – potentially for life.
Despite their limitations, the use of ESCs has persisted in therapeutic development – based largely on the assumption that other types of stem cells were impractical, or otherwise in short supply. However, as it turned out, there were stem cells hidden in plain sight all along.
In 2006, scientists Kazutoshi Takahashi and Shinya Yamanaka demonstrated stem-cell differentiation could be reversed.2 With the right steps and conditions, scientists can take a skin cell, muscle cell, or virtually any other somatic cell type from the body, and coax it back to its prior stem-cell state. This process generates cells called induced pluripotent stem cells, or iPSCs. Unlike embryonic stem cells, iPSCs can be produced on a personalised, as-needed and cGMP-compliant basis. The process can yield a renewable stockpile of cells for everything from CAR-T cancer therapies to tissue and organ transplants. iPSCs can also be made from a variety of sources: either created from a patient’s own cells as autologous therapies, or from donor cells as part of ‘off-the-shelf’ allogeneic treatments.
Early approaches to iPSC reprogramming
Demonstrating that iPSCs could be derived from tissue-specific somatic cells was a pivotal milestone for the field. But before we could hope to use iPSCs to regenerate tissue or treat complex diseases, we were still missing something – a core understanding of the complex epigenetic changes required for precise control of cell differentiation.
It is a two-way street: to start, the differentiated cell (eg, skin cell) must be reverse engineered into an iPSC. But to serve as a viable therapy, the iPSC must then be guided towards its new fate – perhaps as a cardiomyocyte or immune cell. This must all be achieved with permanency and while maintaining the health of the cell.
Some early reprogramming success has been seen with chemically induced pluripotent stem cells,3 using a specific cocktail of chemicals and growth factors to trigger a desired cell fate. Early results for one three-participant trial look good – an exciting signal to the field. But questions remain over the wide-spread efficacy, reproducibility and scalability of chemically differentiated stem cells. Chemical methods can lack specificity, which may produce heterogeneous cell types, or even damage cells and reduce their long-term viability.4 At best, it seems, chemical cocktails represent a messy, indirect route to controlling cell fate.
Enter epigenetics…
Broadly speaking, epigenetics refers to the system of proteins and marks that sits atop our genome – a system that modifies gene expression, guides cell differentiation, and functionally organises our DNA. Any study of the genome is incomplete without considering the epigenomic framework within which genes are read and regulated.
The fields of genomics and epigenetics are so closely linked that a breakthrough in one will often catalyse progress in the other. A prime example of this is DNA binding proteins, such as those found in the CRISPR-Cas system – made famous by 2020 Nobel Prize winners Emmanuelle Charpentier and Jennifer Doudna.5 As new levels of precision in gene targeting are achieved, our ability to accurately shape epi-genetic changes also improves. When non-cutting (aka, nuclease-null) DNA binding proteins like dCas9 are fused to epigenetic effector proteins, they enable precise epigenetic modifications6 to any genomic region, while leaving the target DNA sequence intact.
As our understanding of epigenetics continues to grow, so does our appreciation for the many benefits epigenetic modifications could bring. The ability to tinker with the epigenome could vastly extend the reach and application of gene and cell therapies, and perhaps create a unique class of regenerative medicines. It seems clear that combined with iPSC technology, epigenetics could unlock a whole new world of therapeutic potential.
Epi-effectors: the best tool for the job
To tap into the full potential of iPSCs, we need to control the epigenetic shepherding of stem cells towards their final, specified cell type. While there are several tools that allow us to alter stem cells, epigenetic effectors (epi-effectors) hold extraordinary promise. In part, this is because epi-effectors are uniquely powerful, versatile tools that allow for fine-tuned alterations to cell expression.
Cell differentiation can be thought of as an expansive decision tree, with many branches and forks. Differentiation is an extremely subtle, nuanced process, and the path a cell follows through these branches is often determined by fairly minor differences in gene expression. Direct editing of the genome and the gross application of transcription factor cocktails are comparatively blunt tools, better suited to large, permanent shifts in cell state. However, epi-editing offers a lighter touch. It can create both temporary and permanent changes, which result in enhanced optionality and a more sophisticated approach to chaperoning stem cells through differentiation.
Epi-effectors are also advantageous for their ability to target multiple genes or gene networks at once. It is rare for a specific, desired cell type to be induced by adjusting the volume of a single gene. Differentiation is more akin to a DJ’s sound board, where many dials must be adjusted in parallel to bring about the desired mix. This deft touch is crucial for iPSC-derived therapeutics, which need to scale by many orders of magnitude while maintaining high consistency and standardisation.
Hitting the right notes
The path a stem cell takes to full differentiation has many critical decision points. To create reliable and scalable iPSC-derived therapies, research teams must compose a kind of epigenetic music, using epi-effectors to play genetic ‘chords’ – in the correct order and with precise intensity – to orchestrate a desired result.
To monitor the efficacy of this process, it is possible to view individual cells to examine how they are responding to any given epi-effector, using a technique known as single-cell sequencing.
You can picture the sequencing process as a kind of cellular concert. First, each cell is given a genetic barcode that allows researchers to identify individual cells and track them through the course of the experiment. Once every cell has a tag, the epigenetic music gets turned on. Epi-effectors are brought in to alter the levels in the mix and the refined control offered by epi-editing allows us to precisely modulate gene expression – akin to how advanced audio systems can individually adjust the volume of different instruments.
Once the epi-effectors have done their job, we can use single-cell sequencing and the genetic barcodes to conduct an ‘exit survey’ for these cellular concert-goers, and analyse what changed in them. The barcodes enable the identification of each cell and to assess how well each cell completed the differentiation process.
But what if you needed more information besides how the cells appeared to change? What if you were also interested in how a given cell behaves once it leaves the concert, or whether the concert was so loud that it gave them hearing loss? Before we can safely administer epi-edited iPSCs to patients, this is the level of detail we need to ensure the health and viability of the final, differentiated cell product. Thus, designing these cellular screens in a precise and cost-effective manner can be quite challenging.
Luckily, equipped with today’s advanced genetic tools (and a fair amount of ingenuity), epigenetic research teams are more than up to the task. When combined with the knowledge gained from other gene and cell therapies, the first epi-edited, iPSC-derived, precision regenerative medicines could be just around the corner.
Epigenetics not only offers a route to creating these products, it offers a pathway to doing so at scale. The first successful application of epi-edited stem cells will likely have a domino effect, leadings to hundreds – perhaps thousands – of therapeutic applications. With epi-editing and iPSCs, almost any cell type is potentially within reach. We just need to find the right epigenetic melody with which to compose them.
References
- Butler PC, Gale EAM. Reversing type 1 diabetes with stem cell–derived islets: a step closer to the dream? Journal of Clinical Investigation [Internet]. 2022 Feb 1;132(3). Available from: https://www.jci.org/articles/view/158305/files/pdf
- Takahashi K, Yamanaka S. Induction of Pluripotent Stem Cells from Mouse Embryonic and Adult Fibroblast Cultures by Defined Factors. Cell [Internet]. 2006 Aug;126(4):663–76. Available from: https://pubmed.ncbi.nlm.nih.gov/16904174/
- Mallapaty S. Stem cells reverse woman’s diabetes — a world first. Nature [Internet]. 2024 Sep 26 [cited 2024 Sep 27]; Available from: https://www.nature.com/articles/d41586-024-03129-3
- Sheng CC, Hao J, Hong CC. Chemically Induced Pluripotent Stem Cells (CiPSCs): A Potential Chemical Biological Breakthrough in Reprogramming? 2014 Jul 4;95–102.
- Charpentier E. THE NOBEL PRIZE IN CHEMISTRY 2020 P O P U L A R S C I E N C E B A C KG R O U N D Genetic scissors: a tool for rewriting the code of life [Internet]. 2020. Available from: https://www.nobelprize.org/uploads/2020/10/popular-chemistryprize2020.pdf
- Ilyas M, Shah Q, Gul A, et al. Advances in CRISPR-Cas systems for epigenetics. Progress in molecular biology and translational science [Internet]. 2024 Jan 1 [cited 2024 Oct 22];185–209. Available from: https://pubmed.ncbi.nlm.nih.gov/39266182/
About the author
Cameron Lee, Principal Scientist at Tune Therapeutics
Related topics
Epigenetics, Genetic Analysis, Induced Pluripotent Stem Cells (iPSCs), Stem Cells
Related organisations
Tune Therapeutics
Related people
Cameron Lee (Tune Therapeutics)