3-D bioprinted tissues as disease-in-a-dish models for drug screening
Posted: 6 December 2017 | Anton Simeonov, Marc Ferrer | No comments yet
More than 90% of drugs that enter clinical evaluation fail to reach approval because of lack of efficacy or unexpected toxicity. This failure rate is in large part due to the use of overly simplistic in-vitro cell-based assays and animal models with limited predictive value…
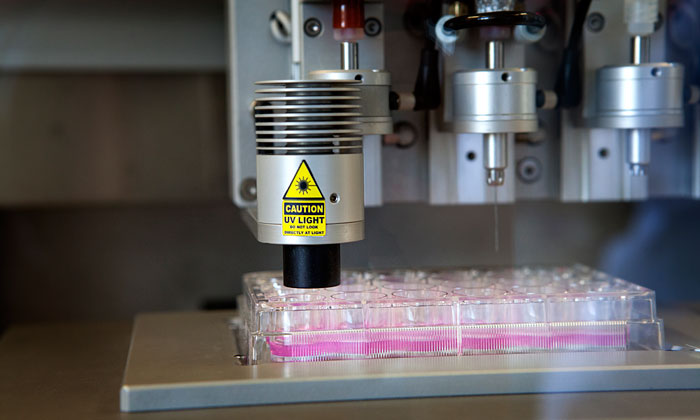
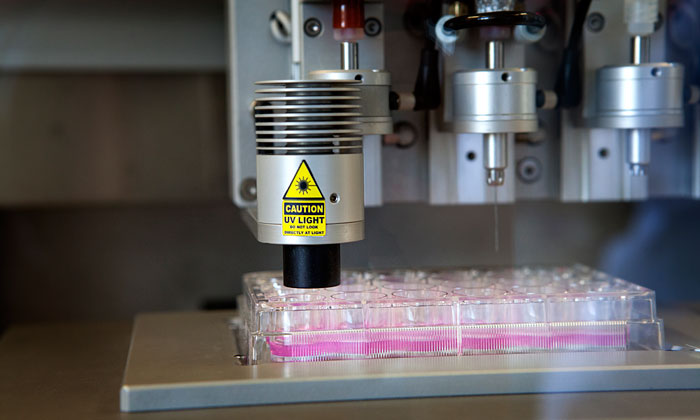
As knowledge of cellular processes in humans and research technologies have improved, it has become more apparent that the complexity of cellular pathways, disease-relevant cellular backgrounds, cell-cell interactions, and cellular-microenvironment interactions in tissues are critical for the understanding of drug efficacy and toxicity in humans, and are considerations that need to be integrated into future drug discovery in vitro cell-based assays.
Three-dimensional (3-D) cell cultures that mimic the spatial organisation of cells in a live tissue in vivo are now being developed to test the activity of therapeutics in possibly more predictive assay systems. These 3-D culture models range from spheres and organoids to bioprinted tissues. Here, we discuss the use of 3-D tissue models for drug screening and, in particular, the use of 3-D bioprinting to fabricate such tissues from the perspective of a laboratory focused on drug screening and discovery.
3-D bioprinting is the process of producing spatially-controlled patterns of functionally viable tissue-relevant cells using a bioprinter. The application of 3-D bioprinting to tissue repair and regenerative medicine to replace damaged tissues and organs has fuelled the recent interest in this field of tissue engineering.3-6 In addition, 3-D bioprinting of human tissues carries the potential to accelerate the drug discovery process by bridging the predictability gap between in vitro and in vivo assay systems and positive clinical outcomes.7-11 The added promise of these 3-D tissue models is that they will reduce animal testing, and perhaps in the future eliminate animal testing, expedite drug development, enhance experimentation capabilities, and save valuable time and funding.
3-D tissue bioprinting promises to address these challenges by integrating five recently emerging technologies:
- 3D bioprinters are now available with precise XYZ alignment to reproducibly fabricate tissues with defined threedimensional geometries
- Biomaterial and biocompatible hydrogels are being developed to support the threedimensional structures of cell assemblies comprising the tissue
- It is also now possible to obtain autologous cells from patients using human induced pluripotent stem cells (hiPSCs) and relevant differentiation protocols, and streamlined processes are being developed to scale up their production
- Microtiter plates are now being designed that enable the delivery of nutrients, mechanical, and chemical cues to the cells seeded in the printed tissues
- The ability to quantitatively characterise the morphology and functionality of printed tissues is also now starting to be possible using both invasive and non-invasive technologies.
Although 3-D printing has been commoditised to the point that 3-D printers are now commercially available at affordable costs, 3-D bioprinters to fabricate tissues are still specialised instruments manufactured by only a few companies. Several bioprinting cell-compatible methods are being used, including laser-assisted,12,13 inkjet,14 and extrusion-based bioprinting.15,16 Extrusion-based bioprinting is perhaps the most popular of the commercially available bioprinters, using either pneumatic micro-extrusion (air-pressure), mechanical micro-extrusion (screw), or solenoid micro-extrusion, because they are compatible with hydrogels of a broad range of viscosities and their reduced shear stress.
Choosing a bioprinter
Bioprinter selection is dictated by the application of interest, whether organ printing for regenerative medicine or developing tissue models for drug testing or screening. For drug screening it is advantageous to have a versatile and modular bioprinter with multiple printing heads for simultaneous use in different bioprinting modes in the same run, in a customisable manner, including micro-extrusion or inkjet, small dead and flexible working volumes, temperature control and UV-crosslinking, to enable different hydrogels that best yield the desired tissue architecture features to be used, different cell dispositions modes and biocompatibility. In addition, easy calibration and positioning of the needles in a variety of different printing vessels, accurate pressure control for reproducible pattern printing, and a computer-assisted design (CAD) software interface that it is easy to use and can create defined spatial patterns, are all desirable.
Bioinks are made up of cells and hydrogel materials that have the right rheological properties of viscosity, low shear stress and biocompatibility to provide the appropriate printability, scaffolding and extracellular material and composition to support tissue structures.17-19 Hydrogels need to provide adequate cell attachment properties, be biodegradable when needed so that cell-secreted extracellular matrix can eventually provide the natural scaffolding and the adequate stiffness for each type of tissue. Cell-laden hydrogels are the most commonly used bioinks because of their adaptability to extrusion-based bioprinting.16 Hydrogel formulations include natural hydrogels such as alginate,20,21 gelatin,22,23 fibrin, collagen, and hyaluronic acid,24,25 as well as synthetic hydrogels such as pluronic hydrogels26 and polyethylene glycol,27 chemically modified variants of both, and in most cases, mixtures are used. The process of choosing the composition of a bioink mixture is based on experimentation and development of compositions with optimal viscosity for printing, shear thinning, stiffness and degradation kinetics, to maintain a printed structure after printing and minimise collapse, control shrinkage and remodelling of the tissue, and biocompatibility, so that cells attach and are viable and functional.28-30
For the purpose of drug discovery, we envisage the development of 3-D bioprinting protocols for the production of a portfolio of tissue models of increasing complexity in microwell plate format, starting with healthy/wild-type cells, for the purpose of initial tissue validation and toxicity testing, and then switching to disease cells by introducing patient primary or iPSC-derived cells in a ‘plugging-in’ modular approach. Protocols for the efficient and automated multi-step cellular maturation of iPSC to the desired differentiated cells are being developed and rigorously standardised and should allow for the robust scale-up in the production of enough cells to yield bioprinted tissues for drug screening.31,32 The use of autologous-iPSC cells will also enable the production of ‘personalised’ tissues. The challenge will remain in finding media conditions in which all the different types of cells in a tissue survive and remain functionally active at the appropriate differentiation stage, and making the relevant cell-cell and cell-stroma interactions.
Characterising fabricated tissues
Methods to quantitatively determine the architecture and function of the fabricated tissues and determine drug effects are key components of drug discovery using 3-D bioprinted tissues. A battery of technologies are being used to characterise the fabricated tissues, both to quickly and efficiently control for consistency upon scale-up, and to quantitate the effect of a drug treatment on a disease phenotype on the tissues. Different technologies with a range of resolution, depth of tissue penetration, and sample processing throughput, are needed. Current determination of the morphology of fabricated tissues relies on histology techniques. Although histology is the gold standard technique for tissue validation during tissue development, the sample processing throughput for this technique is too low to use in a drug discovery setting, whether it is for quality control (QC) of tissue production or for compound screening.
Fluorescence microscopy techniques provide high resolution and the sample processing speed needed for screening and are routinely used in high-throughput screening for drug discovery. However, despite the broad use of confocal microscopy, imaging of tissues poses the challenge of limited depth of penetration.33 Light sheet microscopy is emerging as a rapid fluorescence technique to enable cellular resolution imaging and depth of penetration.34 Nevertheless, improved imaging techniques that are non-destructive, non-invasive and with deep tissue penetration are needed.33 In this regard, imaging technologies such as optical coherence tomography (OCT)35-37 and reflectance confocal microscopy38 are examples of technologies already used in the clinic for tissue imaging and might be adapted to tissue imaging in vitro. Other technologies based on mass spectrometry, such as MALDI,39-41 are also being applied to the detection of biological molecules in tissues slices and producing images of tissue distribution of molecules that are challenging to detect by immunostaining, such as lipids42,43 or glycoproteins,44,45 as well as to study the penetration of small molecules.46,47
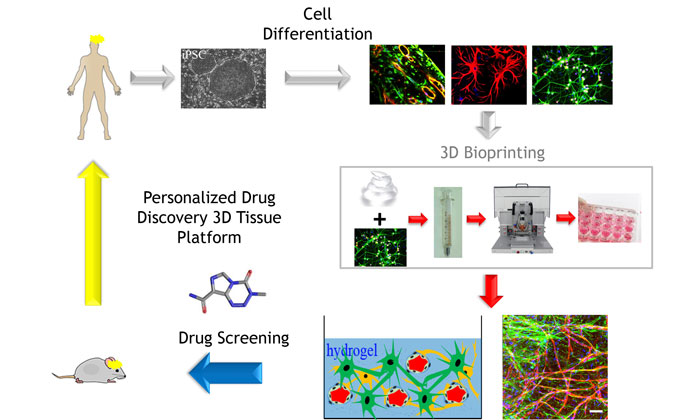
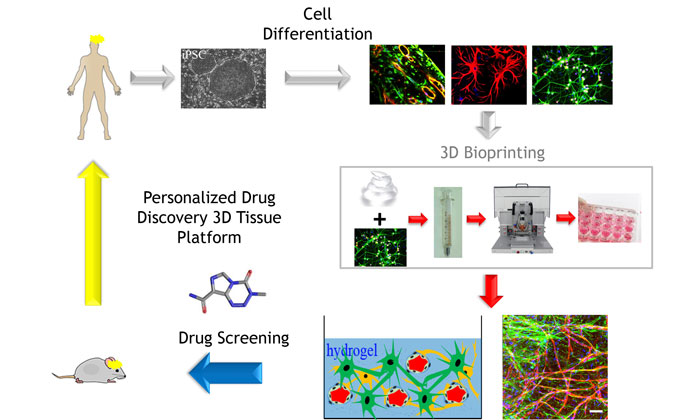
Figure 1: A personalised FIGURE 1 medicine circle for drug discovery where 3-D tissue models provide more clinically relevant data during the different stages of drug discovery and development. Figure generated by Min Jae Song and Marc Ferrer (NIH).
There is a need to develop robust and pharmacologically validated new alternative cell-based models of diseases to enable large-scale compound testing for drug discovery and development. 3-D bioprinted tissue models are being developed as native-like disease-in-a-dish assays for more predictive efficacy and toxicology testing. Focus on technical standardisation, rigorous QC, reproducibility, pharmacological benchmarking, and thoughtful integration and sharing of the results so that the knowledge derived from different improvements can be used as guiding principles by the drug screening and development community in a cost-effective and logical manner is critical. Increased complexity of the bioprinted tissues including disease iPSC-derived cells, vasculature,48-52 and inclusion of immune cells to develop immunocompetent models will further increase the physiological disease relevance of these tissue models. A truly personalised medicine circle for drug discovery as depicted in Figure 1, where a battery of 3-D tissue models provide more clinically relevant data during the different stages of drug development, is now possible and will likely become a reality in the near future.
Biographies
References:
- Harrison RK. Phase II and phase III failures: 2013-2015. Nat Rev Drug Discov. 2016;15(12):817-818.
- Horvath P, et al. Screening out irrelevant cell-based models of disease. Nat Rev Drug Discov. 2016;15(11):751-769.
- Zhang YS, et al. 3D Bioprinting for Tissue and Organ Fabrication. Ann Biomed Eng. 2017;45(1):148-163.
- Sigaux N, et al. Is 3D Bioprinting the Future of Reconstructive Surgery? Plast Reconstr Surg Glob Open. 2017;5(3):e1246.
- Aljohani W, et al. Bioprinting and its applications in tissue engineering and regenerative medicine. Int J Biol Macromol. 2017.
- Murphy SV and Atala A. 3D bioprinting of tissues and organs. Nat Biotechnol, 2014;32(8):773-85.
- Memic A, et al. Bioprinting technologies for disease modeling. Biotechnol Lett. 2017;39(9):1279-1290.
- Peng W, Unutmaz D, Ozbolat IT. Bioprinting towards Physiologically Relevant Tissue Models for Pharmaceutics. Trends Biotechnol. 2016;34(9):722-32.
- Ozbolat IT, Peng W, Ozbolat V. Application areas of 3D bioprinting. Drug Discov Today. 2016;21(8):1257-71.
- Arslan-Yildiz A, et al. Towards artificial tissue models: past, present, and future of 3D bioprinting. Biofabrication. 2016;8(1):014103.
- Holmes AM, et al. Rising to the challenge: applying biofabrication approaches for better drug and chemical product development. Biofabrication. 2017;9(3):033001.
- Guillotin B, et al. Laser assisted bioprinting of engineered tissue with high cell density and microscale organization. Biomaterials. 2010;31(28):7250-6.
- Devillard R, et al. Cell patterning by laser-assisted bioprinting. Methods Cell Biol. 2014;119:159-74.
- Gudapati H, Dey M Ozbolat I. A comprehensive review on droplet-based bioprinting: Past, present and future. Biomaterials. 2016;102:20-42.
- Ozbolat IT, Hospodiuk M. Current advances and future perspectives in extrusion-based bioprinting. Biomaterials. 2016;76:321-43.
- Panwar A, Tan LP. Current Status of Bioinks for Micro-Extrusion-Based 3D Bioprinting. Molecules. 2016;21(6).
- Ji S, Guvendiren M. Recent Advances in Bioink Design for 3D Bioprinting of Tissues and Organs. Front Bioeng Biotechnol. 2017;5:23.
- Hospodiuk M, et al. The bioink: A comprehensive review on bioprintable materials. Biotechnol Adv. 2017;35(2):217-239.
- Skardal A, Atala A. Biomaterials for integration with 3-D bioprinting. Ann Biomed Eng. 2015;43(3):730-46.
- Jia J, et al. Engineering alginate as bioink for bioprinting. Acta Biomater. 2014; 10(10):4323-31.
- Axpe E, Oyen ML. Applications of Alginate-Based Bioinks in 3D Bioprinting. Int J Mol Sci. 2016;17(12).
- Bertassoni LE, et al. Direct-write bioprinting of cell-laden methacrylated gelatin hydrogels. Biofabrication. 2014;6(2):024105.
- Liu W, et al. Extrusion Bioprinting of Shear-Thinning Gelatin Methacryloyl Bioinks. Adv Healthc Mater. 2017: 6(12).
- Pescosolido L, et al. Hyaluronic acid and dextran-based semi-IPN hydrogels as biomaterials for bioprinting. Biomacromolecules. 2011;12(5):1831-8.
- Law N, et al. Characterisation of hyaluronic acid methylcellulose hydrogels for 3D bioprinting. J Mech Behav Biomed Mater. 2017;77:389-399.
- Muller M, et al. Nanostructured Pluronic hydrogels as bioinks for 3D bioprinting. Biofabrication. 2015.7(3):035006.
- Gao G, et al. Bioprinting Cartilage Tissue from Mesenchymal Stem Cells and PEG Hydrogel. Methods Mol Biol. 2017;1612:391-398.
- Holzl K, et al. Bioink properties before, during and after 3D bioprinting. Biofabrication. 2016;8(3):032002.
- Wu Z, et al. Bioprinting three-dimensional cell-laden tissue constructs with controllable degradation. Sci Rep. 2016;6:24474.
- Ribeiro A, et al. Assessing bioink shape fidelity to aid material development in 3D bioprinting. Biofabrication. 2017.
- Singec I, Simeonov A. Translating Stem Cell Biology Into Drug Discovery. Drug Target Rev. 2016;3(2):34-38.
- Pamies D, et al. Good Cell Culture Practice for stem cells and stem-cell-derived models. ALTEX. 2017; 34(1):95-132.
- Bardsley K, et al. Current State-of-the-Art 3D Tissue Models and Their Compatibility with Live Cell Imaging. Adv Exp Med Biol. 2017;1035:3-18.
- Andilla J, et al. Imaging tissue-mimic with light sheet microscopy: A comparative guideline. Sci Rep. 2017;7:44939.
- Fan S, et al. Whole eye segment imaging and measurement with dual-channel spectral-domain OCT. Ophthalmic Surg Lasers Imaging Retina. 2015;46(2):186-94.
- Zhao Q, et al. Evaluation of ultrasound and glucose synergy effect on the optical clearing and light penetration for human colon tissue using SD-OCT. J Biophotonics. 2014;7(11-12):938-47.
- Sun Y, et al. Noninvasive imaging and measurement of accommodation using dual-channel SD-OCT. Curr Eye Res. 2014;39(6):611-9.
- Pellacani G, et al. The impact of in vivo reflectance confocal microscopy for the diagnostic accuracy of melanoma and equivocal melanocytic lesions. J Invest Dermatol. 2007;127(12):2759-65.
- Seeley EH, Caprioli RM. MALDI imaging mass spectrometry of human tissue: method challenges and clinical perspectives. Trends Biotechnol. 2011;29(3):136-43.
- Schwamborn K, Caprioli RM, MALDI imaging mass spectrometry–painting molecular pictures. Mol Oncol, 2010;4(6):529-38.
- Gessel MM, Norris JL, Caprioli RM. MALDI imaging mass spectrometry: spatial molecular analysis to enable a new age of discovery. J Proteomics. 2014;107:71-82.
- Berry KA, et al. MALDI imaging of lipid biochemistry in tissues by mass spectrometry. Chem Rev. 2011;111(10):6491-512.
- Angel PM, et al. MALDI Imaging Mass Spectrometry as a Lipidomic Approach to Heart Valve Research. J Heart Valve Dis. 2016;25(2):240-252.
- Drake RR, et al. MALDI Mass Spectrometry Imaging of N-Linked Glycans in Cancer Tissues. Adv Cancer Res. 2017;134:85-116.
- Angel PM, et al. MALDI Imaging Mass Spectrometry of N-glycans and Tryptic Peptides from the Same Formalin-Fixed, Paraffin-Embedded Tissue Section. Methods Mol Biol. 2017.
- Liu X, Weaver EM, and Hummon AB. Evaluation of therapeutics in three-dimensional cell culture systems by MALDI imaging mass spectrometry. Anal Chem. 2013;85(13):6295-302.
- Lukowski JK, Weaver EM, Hummon AB. Analyzing Liposomal Drug Delivery Systems in Three-Dimensional Cell Culture Models Using MALDI Imaging Mass Spectrometry. Anal Chem. 2017;89(16):8453-8458.
- Zhu W, et al. Direct 3D bioprinting of prevascularized tissue constructs with complex microarchitecture. Biomaterials. 2017;124:106-115.
- Zhang YS, Pi Q, van Genderen AM. Microfluidic Bioprinting for Engineering Vascularized Tissues and Organoids. J Vis Exp. 2017;(126).
- Datta P, Ayan B, Ozbolat IT. Bioprinting for vascular and vascularized tissue biofabrication. Acta Biomater. 2017;51:1-20.
- Kolesky DB, et al. Three-dimensional bioprinting of thick vascularized tissues. Proc Natl Acad Sci U S A. 2016;113(12):3179-84.
- Jia W, et al. Direct 3D bioprinting of perfusable vascular constructs using a blend bioink. Biomaterials. 2016;106:58-68.
Related topics
Screening
Related organisations
National Center for Advancing Translational Sciences' (NCATS)
Related people
Anton Simeonov, Marc Ferrer