Label-free technologies for monitoring drug interactions
Posted: 9 April 2015 | Jean-Francois Masson (Universite de Montreal and Centre for Self-Assembled Chemical Structures), Kristy McKeating (Universite de Montreal)
In his fascinating overview of label-free analysis, Dr. Masson introduces the four main label-free methods: physical, electrochemical, mass-based and optical techniques, before providing the reader with in-depth insight into the most common label-free technique within the pharmaceutical industry: surface plasmon resonance (SPR)…
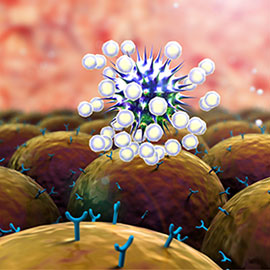
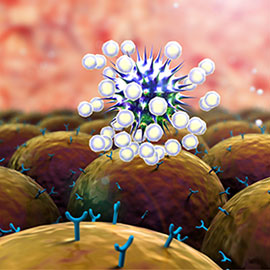
The ability to monitor a bio-molecular interaction is essential in the bioscience field as it enables the study of numerous biological processes such as DNA hybridisation, protein binding, and substrate-enzyme interactions. Additionally, the ability to monitor the interaction of drugs with their specific target receptor is of great importance, particularly in the pharmaceutical industry. Currently, most biochemical assays designed to monitor these types of interactions require the use of mass, optical or radio-labels that may alter the chemical nature of the molecule being studied. This can result in distorted binding, which can lead to erroneous data that is not representative of the real biological interaction. As such, the field of label-free sensing has grown rapidly in recent years in order to provide accurate molecular interaction data from native binding partners. This report provides a brief overview of current label-free technologies, and highlights current state-of-the-art techniques for label-free monitoring of drug interactions.
Jean-François Masson, Associate professor at the University of Montreal Department of Chemistry, discusses the key advantage of label-free technologies, in his article ‘Label-free technologies for biomolecular interaction monitoring’, as enabling the monitoring of bio-molecular interactions with native binding partners, without interference from modified analytes. This, in turn, leads to more accurate biochemical data.
In his fascinating overview of label-free analysis, Dr. Masson introduces the four main label-free methods: physical, electrochemical, mass-based and optical techniques, before providing the reader with in-depth insight into the most common label-free technique within the pharmaceutical industry: surface plasmon resonance (SPR). SPR can detect and quantify a wide array of biomolecules and their interactions and has been employed to great effect for identifying drug molecules that bind to a targeted receptor.
Current label-free technologies
The field of label-free technologies has exponentially grown in the past decade. This is primarily due to the advent of new methodologies and techniques that have found applications in biomarker discovery and drug development in addition to biomolecule sensing and interaction monitoring. Currently valued at around $300 million per year, Frost & Sullivan predicted that the label-free technology market would continue to grow by 15% annually.1 The commercialisation of several technologies has led to the widespread use of label-free techniques in academic, government and industrial labs, with the pharmaceutical industry being the most prevalent.2
For clarification, in this report, a label-free technology refers to a method of measurement that does not require chemical or isotopic modification of the analyte, substrate or binding partner for quantification, identification or affinity measurement. This definition of label-free includes techniques that require the immobilisation of a molecular receptor, capture agent or a bio-molecular binding partner to a substrate (i.e. an antibody bound to the well of an ELISA plate would still be considered label-free).
Common chemical or isotopic modifications involve the addition of mass tags, radiolabels or fluorescence tags to the molecule being investigated.3 While effective in many applications, the labelling process can alter the chemical properties of the molecules, create steric hindrance within the binding site or involve relatively complex synthetic steps and procedures. The major advantage of label-free technologies, therefore, lies in the ability to monitor bio-molecular interactions with native binding partners, without interference from modified analytes, leading to more accurate biochemical data. Additionally, several label-free techniques can monitor the response in real-time, enabling accessible kinetic information about association or dissociation. These advantages of label-free techniques help to explain their popularity in modern biomedical, pharmaceutical and analytical sciences.
The plethora of label-free techniques can be categorised into four main methods of analysis. Physical methods of analysis include microcalorimetry and acoustic resonance measurements,3 while electrochemical methods involve amperometric, potentiometric, and impedance spectroscopy4 which utilise electrodes and nanomaterials, including nanowires, nanotubes, and nanopores.5 Mass-based methods of analysis include mass spectrometry,6 quartz crystal microbalance measurements,7 and microcantilevers.8 Finally, optical techniques include waveguides, plasmonics and molecular spectroscopy.9-13 Optical methods of analysis constitute a large market share of the label-free technology industry, with several commercialised techniques exploiting the use of surface plasmons, interferometry, photonic crystals and molecular spectroscopy.10 A variety of techniques based on ring resonators and whispering gallery modes,14 nanoplasmonics and surface enhanced spectroscopies15 are currently being developed to further improve upon the performance of label-free techniques.
Monitoring drug interactions by surface plasmon resonance
Surface plasmon resonance (SPR) is currently the most common label-free technique used in the pharmaceutical industry.2 SPR sensing relies on changes in the refractive index of an aqueous media near a thin metal film, most commonly gold, which is indirectly related to the mass of molecules in solution. As a result, SPR can detect and quantify a wide array of biomolecules and their interactions via a local change in refractive index, including those between a drug molecule and its receptor. SPR has been employed to great effect for identifying drug molecules that bind to a targeted receptor and several examples have been reported in scientific literature. 2,12,16
A major application of SPR is in the advancement of high-throughput drug screening (HTS), a well-established method in the pharmaceutical industry for screening large libraries of compounds for successful interactions with biological targets.17 In recent years SPR has found widespread use in the screening of both drugs and drug fragments by binding a receptor for which drugs are being developed to the SPR sensor chip, and measuring the interactions of a vast number of compounds.18 Binding of a soluble drug receptor can be carried out using well-developed chemical reactions or affinity capture methods,19 however, membrane receptors have proven to be more difficult to analyse by SPR.16,20 Since these membrane receptors remain one of the principal targets for new drug discovery, many methods have been developed in order to combat this problem.16 The most common of these solutions include the use of biomimetic SPR sensors, usually liposome functionalised sensors, that enable bimolecular analysis and kinetic studies of potential new drugs for membrane bound receptors.9,21,22 In addition, SPR imaging has recently found favour in drug discovery as it enables the simultaneous and multiplexed detection of thousands of biochemical interactions, providing real-time kinetic data within a few minutes, and therefore has great potential within the pharmaceutical industry. 23,24
One of the main issues associated with drug interaction monitoring via SPR is low sensitivity due to the relatively small molecular weight of the analytes involved. To overcome this problem, many commercial instruments have high quality optics and complex fluidics installed, as well as automatic data processing, in order to maximise the response obtained from drug molecules binding to their receptors. Despite the increased cost of acquisition resulting from these modifications, SPR sensing remains the gold standard for affinity measurements of drugs with biological receptors. The sensitivity can also be improved by binding the drug in an array format on the SPR sensor, while the receptor is injected, and the interaction with surface bound drugs is monitored. This approach offers greater sensitivity as the receptor carries a larger mass and refractive index change, however this methodology requires a soluble receptor, and also requires modification of the drug, and so loses the inherent advantages of a label-free method of analysis. Alternatively, the sensitivity of SPR sensing can easily be improved with allosteric receptors. The change in conformation of receptors near the surface of the SPR sensor leads to a notable change in refractive index induced by the binding process of the drug, which is detected by the SPR sensor.19,25
Although the analysis of a drug binding to its receptor provides valuable information about binding kinetics, this may not necessarily translate to drug efficacy. Thus, studies need to be conducted with the cells targeted by the therapeutic drugs to provide information closer in nature to the interaction that will take place inside a living organism. Cell layers can be cultured directly on the SPR chip,26,27 and the exposition of cells to potential therapeutic drugs can lead to a change in cell morphology, such as contraction or spreading, as well apoptosis.28,29 This will result in a refractive index change at the interface between the cell and metal surface,28 and thus SPR sensing can monitor the interaction of the drug with the cell in a label-free and non-invasive manner.12 27,30 Improvements upon traditional SPR methods for cellular analysis include the development of long-range SPR (LRSPR) sensors that can enhance detection depths inside living cells,31 as well as coupling of SPR to other well-known techniques such as impedance spectroscopy32 and Fourier Transform infrared spectroscopy (FTIR-SPR).33 Additionally, with the recent advent of SPR microscopy (SPRM), the binding kinetics of membrane proteins on living cells can be measured in their true native environment.34,35
SPR for therapeutic drug monitoring
While SPR sensing has significantly contributed to drug development by enabling the measurement of the binding kinetics of therapeutic drugs with their receptors, SPR sensing is also quantitative and can measure drug concentrations in bio-fluids. Therapeutic drug monitoring (TDM) aims to provide accurate measurements of the bioavailable level of a drug in the blood of patients, allowing for personalised drug dosing according to patient specific metabolism.This is particularly important for drugs with a narrow therapeutic window, as finding the optimum drug concentration is of the utmost importance to a patient’s health and safety. There is also a need for decentralisation of biochemical techniques in an attempt to move towards point-of-care analysis, allowing for rapid turnaround times in monitoring important biomarkers and drug levels in patient samples.
SPR instruments can be miniaturised, creating a portable and highly sensitive analysis format. Recently, an SPR assay was developed to detect therapeutic drugs, exploiting the competition of the drug with a nanoparticle functionalised with a co-substrate of the molecular receptor. This competitor molecule must show an affinity for the receptor and possess a method for being immobilised on nanoparticles.36 Generally, this competitor can be a different molecule identified during development that did not make it through to the final stages of the drug development program. The molecular receptor targeted by the drug is immobilised on the SPR sensor, and the therapeutic drug being analysed competes for this receptor with the functionalised nanoparticles. In the absence of any drug molecule, a high SPR response is obtained resulting from the interaction of the high mass nanoparticles with the surface-bound receptor. Upon introduction of the therapeutic drug, which preferentially binds to the surface, the signal obtained from the nanoparticles will drop depending on concentration as the sites available for nanoparticle interaction are reduced. This concept was successfully demonstrated for methotrexate,37 providing a response time of only one minute, and it is predicted that this analysis format, pending on the availability of the molecular receptor and competitor, could be widely applicable for a variety of therapeutic drugs.
TDM is particularly important for drugs where dosage can be critical, and the ability to detect these drugs in a rapid and sensitive manner at the point of care could drastically improve patient treatment. Additionally, TDM could decrease the risk of adverse effects resulting from toxic drugs, which could facilitate approval by the US Food and Drugs Administration. This concept of combining therapy and diagnostics is becoming increasingly popular, and this relatively novel field of theranostics could greatly benefit from label-free technologies such as SPR.
Perspective
Surface plasmon resonance sensing provides a simple and effective method of monitoring drug interactions in a label-free manner using real-time analysis. These advantages have been exploited over the years within the pharmaceutical industry in order to improve upon methods of drug discovery, allowing for new methods of high-throughput drug screening. As the pharmaceutical industry has advanced, so has the nature of SPR sensing and recent developments, including the advent of SPR imaging, are helping to further progress this field enabling multiplexed analysis of numerous biochemical reactions.
In recent years, SPR has moved from the analysis of soluble receptors towards the analysis of membrane-bound receptors, which are key in the development of new therapeutic drugs. Additionally, the ability to analyse live cells is of great importance in gathering information on drug interaction in a native environment. The coupling of SPR to other well-established analytical techniques is becoming increasingly popular and will no doubt advance the field, improving upon sensitivity and selectivity, while enabling analysis in crude fluids, effectively abolishing sample pre-treatment. There are still areas of drug analysis that require a solution, for example, designing a label-free method for monitoring drug and molecular transport through the blood brain barrier is highly desirable, and only through further development of label free technologies will this become a reality. In a discipline that is currently moving towards patient-specific treatment and with the emerging field of theranostics, it is clear that the future of the pharmaceutical industry will rely heavily on the development of label-free technologies such as SPR.
References
- http://www.drugdiscovery.frost.com, 2012.
- Olaru, A. Bala, C., Jaffrezic-Renault, N., Aboul-Enein, H. Y. Critical Reviews in Analytical Chemistry 2014, 45, 97.
- Cooper, M. Analytical and Bioanalytical Chemistry 2003, 377, 834.
- Xi, B., Yu, N., Wang, X., Xu, X., Abassi, Y. Biotechnology Journal 2008, 3, 484.
- Huang, Y. X., Chen, P. Advanced Materials 2010, 22, 2818.
- Roddy, T. P., Horvath, C. R., Stout, S. J., Kenney, K. L., Ho, P.-I., Zhang, J.-H., Vickers, C., Kaushik, V., Hubbard, B., Wang, Y. K. Anal. Chem. 2007, 79, 8207.
- Cheng, C. I., Chang, Y. P., Chu, Y. H. Chem. Soc. Rev. 2012, 41, 1947.
- Ji, H.-F., Gao, H., Buchapudi, K. R., Yang, X., Xu, X., Schulte, M. K. Analyst 2008, 133, 434.
- Danelian, E., Karlén, A., Karlsson, R., Winiwarter, S., Hansson, A., Löfås, S., Lennernäs, H., Hämäläinen, M. D. J Med Chem 2000, 43, 2083.
- Li, M., Cushing, S. K., Wu, N. Analyst 2015, 140, 386.
- Mariani, S., Minunni, M. Analytical and Bioanalytical Chemistry 2014, 406, 2303.
- Yanase, Y., Hiragun, T., Ishii, K., Kawaguchi, T., Yanase, T., Kawai, M., Sakamoto, K., Hide, M. Sensors 2014, 14, 4948.
- Bolduc, O. R., Masson, J.-F. Anal. Chem. 2011, 83, 8057.
- Vollmer, F., Arnold, S. Nat Meth 2008, 5, 591.
- Pinzaru, S. C., Pavel, I., Leopold, N., Kiefer, W. Journal of Raman Spectroscopy 2004, 35, 338.
- Patching, S. G. Biochimica et Biophysica Acta 2014, 1838, 43.
- Mayr, L. M., Bojanic, D. Current Opinion in Pharmacology 2009, 9, 580.
- Pröll, F., Fechner, P., Proll, G. Analytical and Bioanalytical Chemistry 2009, 393, 1557.
- Bolduc, O. R., Lambert-Lanteigne, P., Colin, D. Y., Zhao, S. S., Proulx, C., Boeglin, D., Lubell, W. D., Pelletier, J. N., Fethiere, J., Ong, H., Masson, J.-F. Analyst 2011, 136, 3142.
- Khandjian, E. W. Bio-Technology 1987, 5, 165.
- Baird, C. L., Courtenay, E. S., Myszka, D. G. Analytical Biochemistry 2002, 310, 93.
- Cooper, M. A. Journal of Molecular Recognition 2004, 17, 286.
- Pillet, F., Romera, C., Trévisiol, E., Bellon, S., Teulade-Fichou, M.-P., François, J-M., Pratviel, G., Leberre, V. A. Sensors and Actuators B 2011, 157, 304.
- Wang, Y., Zhang, C., Zhang, Y., Fang, H., Min, C., Zhu, S., Yuan, X. C. Analytical Methods 2015.
- Gestwicki, J. E., Hsieh, H. V., Pitner, J. B. Anal. Chem. 2001, 73, 5732.
- Chen, K., Obinata, H., Izumi, T. Biosensors & Bioelectronics 2010, 25, 1675.
- Robelek, R., Wegener, J. Biosensors & Bioelectronics 2010, 25, 1221.
- Chabot, V., Cuerrier, C. M., Escher, E., Aimez, V., Grandbois, M., Charette, P. G. Biosensors and Bioelectronics 2009, 24, 1667.
- Nishijima, H., Kosaihira, A., Shibata, J., Ona, T. Anal. Sci. 2010, 26, 529.
- Mizuguchi, T., Uchimura, H., Kataoka, H., Akaji, K., Kiso, Y., Saito, K. Analytical Biochemistry 2012, 420, 185.
- Chabot, V., Miron, Y., Grandbois, M., Charette, P. G. Sens. Actuator B-Chem. 2012, 174, 94.
- Michaelis, S., Wegener, J., Robelek, R. Biosensors & Bioelectronics 2013, 49, 63.
- Ziblat, R., Lirtsman, V., Davidov, D., Aroeti, B. Biophys. J. 2006, 90, 2592.
- Campbell, C. T., Kim, G. Biomaterials 2007, 28, 2380.
- Campbell, N., Reece, J. Biology, 6 ed., Benjamin Cummings: San Francisco.
- Zhao, S. S., Bichelberger, M. A., Colin, D. Y., Robitaille, R., Pelletier, J. N., Masson, J.-F. Analyst 2012, 137, 4742.
- Zhao, S. S., Bukar, N., Toulouse, J. L., Pelechacz, D., Robitaille, R., Pelletier, J. N., Masson, J.-F. Biosensors and Bioelectronics 2015, 64, 664.
Biographies
Jean-François Masson is an Associate Professor of Chemistry at the Université de Montréal. His expertise encompasses biosensing with plasmonic materials, instrument development, surface chemistry to minimise biofouling and detection of proteins and drugs directly in crude biofluids. Jean-François holds a BSc in Chemistry from Université de Sherbrooke (2001), a PhD in Analytical Chemistry from Arizona State University (2005), and a post-doctoral fellowship at Georgia Tech (2005-2007). Jean-Francois has received several awards including the Tomas Hirschfeld award (2005), the Fred Beamish award (2013), an Alexander von Humboldt fellowship (2013-2014), and a CNC-IUPAC travel award (2015). He has published more than 50 research articles and he is an Associate Editor for the Analyst. His research has led to filing eight patents on diverse instrumental, materials or surface chemistry innovations for biosensing.
Kristy McKeating obtained her PhD in 2013 from the University of Strathclyde in Glasgow, working under the supervision of Dr. Karen Faulds on the development of novel nanosensors for molecular diagnostics, specialising in enhanced Raman spectroscopies. She is currently a postdoctoral researcher at the University of Montreal working within the group of Prof. Jean-François Masson to develop functionalised nanomaterials for the analysis of clinically-relevant biomolecules in complex matrices using surface plasmon resonance.
Related topics
Drug Discovery, Label-Free
Related organisations
Centre for Self-Assembled Chemical Structures, University of Montreal
Related people
Jean-Francois Masson, Kristy McKeating