Transforming drug discovery, development and delivery with flow chemistry
Posted: 11 April 2019 | Dr Omar Jina - Syrris Ltd, Prof Nicola Tirelli - Italian Institute of Technology Central Research Lab Genova | No comments yet
Flow chemistry is on the rise thanks to the commercial availability of benchtop continuous flow systems that has driven widespread adoption of the technique across a broad range of applications in both academia and industry. In particular, flow chemistry is delivering significant benefits in the area of drug discovery and development by enabling chemistry to be performed that is not currently achievable using traditional techniques.
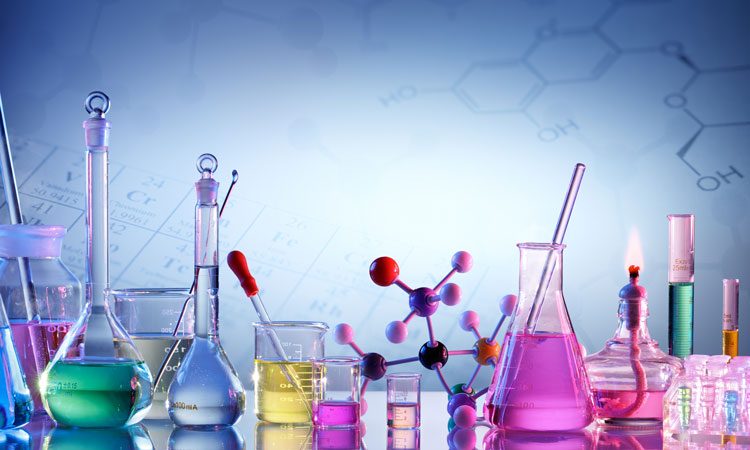
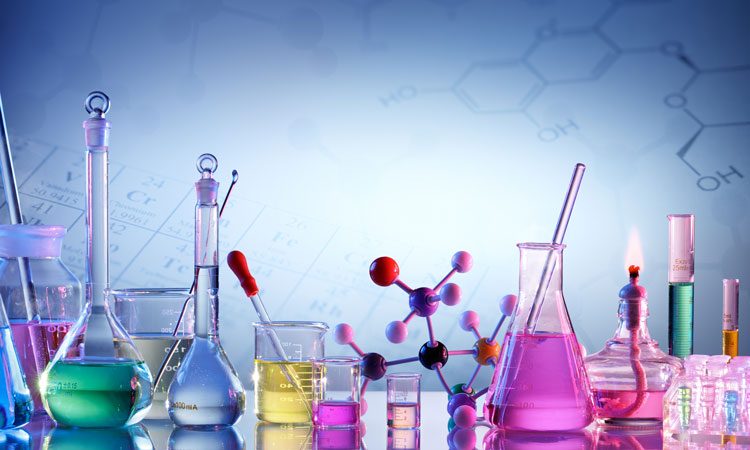
The benefits of flow chemistry are seen throughout all areas of the pharmaceutical pipeline, from library synthesis in discovery to rapid reaction optimisation in development. Most recently, there is huge interest in using continuous processes for drug delivery and release, due to their ability to make extremely reproducible nano‑ and microparticles. The principle of flow chemistry is most easily understood when compared with batch chemistry – the traditional approach for chemical reactions. Batch chemistry involves loading reagents into a single container – often a round bottom flask or jacketed reactor vessel – while heating and stirring to ensure the reaction proceeds to completion, with subsequent separate steps required for work-up and product purification. In contrast, in flow chemistry, reagents are continuously pumped through a temperature-controlled tube, pipe or microfluidic chip, and the generated product can be collected as the reaction proceeds. A combination of several factors – such as reactor geometry, enhanced temperature control and rapid, efficient mixing – offers excellent reaction control and enhanced reproducibility.
Exploring new frontiers
Transferring batch chemistry reactions to a flow regime has opened new reaction possibilities that simply could not be achieved with conventional techniques; allowing scientists to explore new chemical spaces and develop previously inaccessible reaction schemes, such as telescoping multi-step reactions. For example, the product of a deprotonation reaction performed at -40°C can flow straight into another reactor at 200°C for nucleophilic addition. This streamlined workflow – almost impossible, or at least laborious, using batch chemistry – potentially opens the door for the exciting discovery of new molecules. Flow chemistry can also easily accommodate other techniques, such as photo- or electrochemistry, with one notable application using flow electrochemistry to directly synthesise metabolites. Once a medicinal chemist has achieved a ‘hit’ compound, the candidate can be dissolved and pumped through a flow electrochemical cell to yield a range of selectively oxidised metabolites. This simple operation eliminates the need to develop new synthetic pathways to produce the metabolites, potentially saving months of time.
Faster and safer
Not every batch process can be translated to a flow regime, but the vast growth in high-quality academic papers published over the last five to 10 years is testament to the rapid adoption of flow chemistry thanks to the unique benefits it offers. The small volume flow reactors are far easier to pressurise than larger batch set-ups, enabling elevated solvent boiling points and higher reaction temperatures, thereby increasing the rate of reaction. In addition, the fact that only a small volume of reagent is reacting at any one time makes the whole process a lot safer, particularly when working with hazardous or highly exothermic reagents. A deciding factor for many scientists investigating flow chemistry systems is the ability to rapidly optimise reaction conditions, reducing costs and saving valuable time. For example, the reaction time, temperature, concentration and reagent ratio can be easily adjusted, allowing multiple reaction conditions to be investigated sequentially with a simple solvent flush required between experiments. This is an ideal workflow for medicinal chemists, who often only require small volumes of product for characterisation and stand to benefit from speeding up discovery pipelines.
Nanoparticle drug delivery
One of the standout benefits of flow chemistry is the enhanced control and reproducibility it offers over equivalent batch processes, particularly in the area of nanoparticle drug delivery. This field has been a subject of interest for over 20 years, due to its advantages over conventional treatment regimes. Encapsulation of an active pharmaceutical ingredient (API) in a polymer carrier particle protects the API from degradation and allows poorly soluble compounds to be used as suitable drug candidates.
The benefits of flow chemistry are seen throughout all areas of the pharmaceutical pipeline, from library synthesis in discovery to rapid reaction optimisation in development
In addition, the nanoparticles can be adapted with functional groups to target specific biological settings, maximising the efficacy of the API while reducing the dose and, as a result, the potential for side effects. The physical properties of these nanoparticles have a direct effect on the pharmaceutical therapeutic effect. Ensuring that the particle size distribution is minimised, the API loading maximised and, above all, that these properties are reproducibly obtained, is critical to controlling the therapeutic dose. Particle size distribution arguably has the biggest implication for drug delivery, as the nanoparticle size determines the rate of diffusion through a tissue and the mechanism of cellular absorption. The subsequent release of the API – usually by simple diffusion or nanoparticle degradation/response – is also strongly dependent on the nanoparticle diameter; the greater surface area to volume ratio of smaller nanoparticles is likely to result in a more rapid release and potentially high API concentrations and associated side effects. Consequently, a broad size distribution leads to poor control over how the API is released, making it harder to determine whether the patient is receiving the required therapeutic dose.
Controlling the particle size distribution also delivers significant upstream advantages. During research and development, working with homogeneous, uniformly-sized particles makes it easier to confidently rationalise biological results and transfer the process to a GLP or GMP environment – a necessary step for clinical translation of the product. Consequently, the development of production methods that both reduce polydispersity and control the average particle size has been a key objective in this field.
Nanoprecipitation
Nanoprecipitation is the most common method for obtaining particles less than a micron in diameter and has traditionally relied on a three-stage process: co-dissolution of a hydrophobic polymer and the API in a water miscible solvent; mixing of this organic phase with an aqueous solution; followed by precipitation of polymer nanoparticles with the encapsulated API. Precipitation is conducted in the presence of surfactants – or using polymers that in themselves are surfactants – to prevent polymer aggregation and ensure distinct nanoparticles are formed. Prior to operating in flow, the only option was to use batch processes, which were conceptually easy to assemble and could produce large volumes of material in a short period of time. One-pot pouring, or dropwise addition of the organic phase to the aqueous solution, is the standard technique for nanoprecipitation, yet this simplicity is undermined by a key disadvantage; it is difficult to set up or scale up a batch process with perfectly reproducible mixing. Even a seemingly inconsequential variable, such as the distance between the magnetic stirrer and the point of injection of the organic phase, can significantly affect the average particle diameter and size dispersity.
From batch to flow
Carrying out nanoprecipitation in microfluidics-based devices offers a solution to this problem. The lack of reproducibility encountered with batch processes is overcome by the superior control offered by the predetermined fluid dynamics of microfluidic channels, which offers a fixed size and geometry. For example, in a cross-shaped, flow-focusing microfluidic chip, the organic phase passes through a central channel and concentrates into the middle region, where water is added laterally via the two remaining perpendicular and counter-flowing channels. Mixing almost invariably takes place under laminar flow, and the connected pumps ensure slow and consistent mixing, making nanoprecipitation a straightforward process to replicate. The size of the precipitated particles is strongly dependent on the aqueous-to-organic ratio, which is easily varied and controlled in a microfluidic process, allowing scientists to create nanoparticles within a specified diameter range. Running several microfluidic chips in parallel can also be used to scale up production, generating over a kilogram of nanoparticles per day.
While flow chemistry’s reproducibility and enhanced control of nanoprecipitation has established it as a reliable alternative to batch chemistry in the field of drug delivery, batch processes still have a complementary role to play. Their simplicity makes them ideal for exploring new materials or experimental conditions, and performing initial screenings without running the risk of, for example, blocking the microfluidic channels. Once nanoprecipitation has been confirmed and refined under batch conditions, it can be transferred to a flow scheme for better reproducibility and control of the average nanoparticle diameter and size distribution.
What’s next for flow chemistry?
The area of nanoparticle drug delivery is only one of many fields that has benefitted from the adoption of flow chemistry. Enhanced control combined with faster, safer reactions and rapid library synthesis make flow chemistry a versatile technique in the world of drug discovery and development. The novel flow technologies now available to academia and industry will continue to be catalysts for innovation and experimentation in the future, offering a desirable alternative to batch processes, opening up new possibilities for multi-step reactions and allowing exploration of previously unattainable chemistry.
Biographies
Related topics
Drug Delivery, Drug Discovery, Nanoparticles