Biosynthetic polysaccharide-based drugs and nutraceuticals from non-animal sources
Posted: 2 December 2020 | Payel Datta (Rensselaer Polytechnic Institute), Robert J Linhardt (Rensselaer Polytechnic Institute) | No comments yet
Glycosaminoglycans (GAGs) are a class of naturally occurring polysaccharides that play vital roles in cellular functions. GAGs (eg, hyaluronic acid, heparosan, chondroitin, chondroitin sulfate, heparan sulfate and heparin) have also been utilised in biopharmaceutical and nutraceutical industries. Animal-sourced GAGs can contain process impurities and contaminants, which may result in adverse effects. To overcome this issue, efforts have focused on the industrial production of animal-component free GAGs. This review focuses on the production of tailored biosynthetic GAGs that have specific and novel functions, including biosynthetic heparins, potential anti-viral and drug delivery applications.
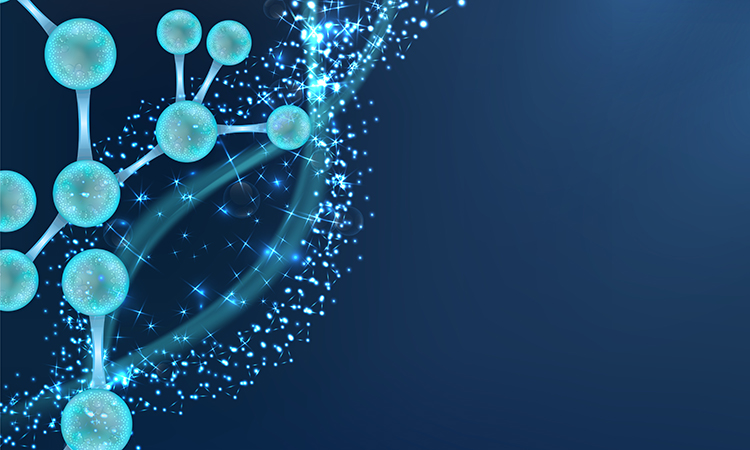
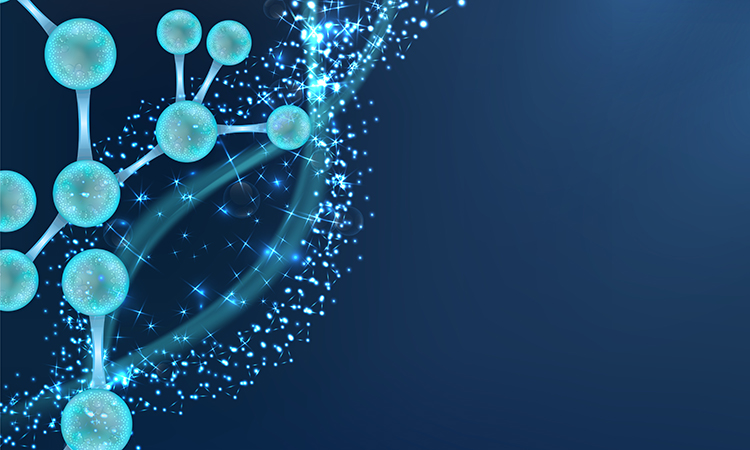
Introduction
GAGs are a family of linear polysaccharides containing repeating disaccharide units of uronic acid and N-acetyl glucosamine (GlcNAc) or N-acetyl galactosamine (GalNAc). Non-sulfated GAGs (eg, hyaluronic acid, heparosan and chondroitin) are biosynthesised in certain prokaryotes. These non-sulfated GAGs are bacterial capsular polysaccharides (CPS) and contribute to the pathogenicity and survival of these bacteria in their host. These non-sulfated GAGs are also produced in animal cells and, in some cases, are the precursor backbones for sulfated GAGs (eg, heparin, heparan sulfate, chondroitin sulfate and dermatan sulfate). For example, heparosan is the non-sulfated linear polysaccharide composed of the repeating disaccharide unit [→4) β-D-glucuronic acid (GlcA) (1→4) α-D-(GlcNAc) (1→]n. The heparosan chain is sequentially modified by tissue-specific sulfotransferases and C5-epimerase to form sulfated heparan sulfate and highly sulfated heparin. Chondroitin, [→4)β-D-GlcA (1→3)α-D-GalNAc) (1→]n, is similarly transformed to chondroitin sulfate or dermatan sulfate. The sulfated domains within these sulfated GAGs serve as the binding sites for specific biological macromolecules, including blood proteins and viral capsular proteins. For instance, heparin is produced chiefly in the mast cells and comprised of highly sulfated pentasaccharide domains that bind to antithrombin III, preventing coagulation. Unfractionated heparins and low molecular weight heparins have been widely used as an anticoagulant drug.
GAGs are present on the cell surface, in the extracellular matrix and form an integral component of the connective tissue systems. Both non-sulfated and sulfated GAGs play vital regulatory roles in biological and cellular functions, including homeostasis, cell growth, development, cardiovascular disease, cancer, infection and wound repair. GAGs have also been widely utilised in the biopharmaceutical and nutraceutical industries:
- Hyaluronic acid and its derivatives are used in a wide range of pharmaceuticals, such as Euflexxa, Healon, Orthovisc, Restylane, Synvisc and Seprafilm. In 2019, the global market for hyaluronic acid was valued at $9.1 billion.
- Both hyaluronan and heparosan are being explored as potential drug delivery vehicles1
- Heparin is a widely used anticoagulant drug with a global market value of $6.49 billion in 2018
- Chondroitin sulfate-type A is a popular dietary supplement and in 2019, the global market for chondroitin sulfate was valued at $1.14 billion.
The conventional industrial production of hyaluronic acid, chondroitin sulfate and heparin relies on animal tissues: hyaluronic acid is extracted from rooster combs and bovine/porcine eyes; chondroitin sulfate is extracted from animal cartilage; and heparin is extracted from porcine/bovine intestine and lung tissues. Animal-sourced products may contain process impurities and contaminants, including transmissible infective agents (prions, viruses, bacteria residues), other polysaccharides, (macro)-molecules, proteins, lipids and nucleic acids. For example, in 2008 heparin contamination/adulteration with over-sulfated chondroitin sulfate led to adverse effects, including death, in patients. The incident demonstrated a need to develop a well-characterised biomanufacturing process and sensitive analytical tools. Additionally, the supply chain of animal-sourced GAGs is unstable and may be impacted by adverse events.
This review article focuses on advances in the production of biosynthetic polysaccharide-based drugs and nutraceuticals from non-animal sources and their potential applications (Figure 1).
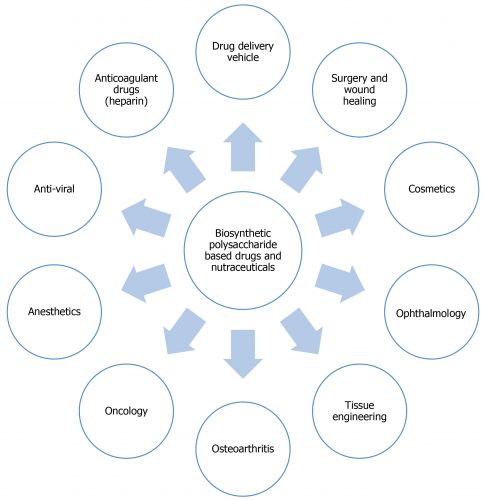
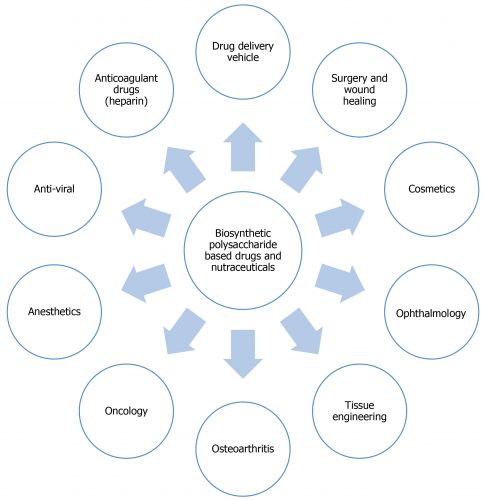
Figure 1: Biosynthetic polysaccharide-based drugs and nutraceuticals
Biosynthetic polysaccharide-based drugs and nutraceuticals from non-animal sources
The biomanufacturing of polysaccharide-based drugs and nutraceuticals has been possible due to advances in strain characterisation methods, bioprocess optimisation tools, sensitive analytical techniques and cell engineering strategies. Strain characterisation tools have empowered the identification of natural microbial producers of simple polysaccharides. Examples of strain characterisation tools include high-coverage, whole-genome sequencing, rapid annotation using a subsystem technology server with Glimmer3 as a gene caller, metabolic reconstruction and comparative genomics analysis.2 Certain simple prokaryotes have the necessary genes to biosynthesise non-sulfated GAGs. For example, E. coli K4 is a natural producer of chondroitin, Streptococcus equi is a natural producer of hyaluronic acid and Pasteurella multocida type D strain, E. coli K5 and E. coli Nissle 1917 are natural producers of heparosan. Analytical methods critical for biomanufacturing of GAGs3-5 include nuclear magnetic resonance (NMR) spectroscopy and liquid chromatography-mass spectrometry (LC‑MS), which can define GAG structure and disaccharide composition. The molecular weight (MW) and polydispersity, critical to quality (CTQs) attributes, rely on gel permeation chromatography. A relatively mono-dispersed MW distribution is a desirable CTQ attribute for downstream processing and applications.
Microbial production of non-sulfated GAGs (hyaluronic acid, heparosan and chondroitin) has been established. One example is the use of high cell fed-batch density fermentation of E. coli K5 in a chemically defined media for heparosan production.5 Non-sulfated GAGs can be further modified using chemicals and enzymes to form highly sulfated GAGs (eg, chondroitin sulfate and heparin). For example, S. equi has been utilised for the production of hyaluronic acid.6 The MW of GAG needs to be modified based on its application. For hyaluronic acid, very highly controlled fermentation parameters and downstream processing results in hyaluronic acid with very high MW. An example of fermentation-derived chondroitin sulfate is Mythocondro®-Gnosis.7 E. coli O5:K4:H4 strain U1-41 (ATCC 23502) has been adapted to high cell density fermentation for the production of non-sulfated chondroitin. Post-fermentation, the chondroitin is purified, processed and chemically modified using regioselective sulfation to produce chondroitin sulfate. An example of fermentation-derived heparin is biosynthetic heparin.8 E. coli K5 (Serovar O10:K5:H4; ATCC #23506) and E. coli Nissle 1917 (Serovar O6:K5:H1) have been adapted to high cell density fermentation for the production of heparosan. The heparosan recovered from fermentation, once purified, represents an important nutraceutical that has the potential to be used as an inert drug delivery vehicle. In addition, purified heparosan can be sequentially chemoenzymatically modified to produce biosynthetic heparin. The heparin biosynthetic enzymes are of mammalian-origin and have been expressed and purified using high cell density fermentation technology. The possibility of using well characterised and controlled chemoenzymatic modification reactions could generate tailored biosynthetic GAGs that have specific and novel functions.4,8-10 For example, biosynthetic heparin and biosynthetic TriS-heparin (an intermediate in the biosynthetic heparin synthesis pathway) show antiviral activity.4,10 Researchers used a competitive surface plasmon resonance (SPR) to evaluate in vitro interactions of sulfated polysaccharides, including biosynthetic heparin and biosynthetic non-anticoagulant TriS-heparin, with the SARS-CoV-2 Spike (S) glycoprotein.
Conclusion
Advances in synthetic biology and metabolic engineering tools have led to research focusing on strain improvements and the creation of in-cell microbial factories that can synthesise tailored polysaccharides with defined functionalities.12 For example, synthetic biology tools have been used to study the heparosan pathway in E. coli to increase the heparosan yield.13,14 The metabolic pathway for GAG synthesis is interspersed with other cellular pathways. For example, glucose is used as the precursor molecule for GAG building blocks as well as cell metabolism. Fine-tuning the metabolic pathway to maintain cell health and growth and increase the desired GAG production is desirable.15,16 Synthetic biology tools have been instrumental in the study and metabolic engineering of microbial and mammalian cells. Examples of synthetic biology tools include: in silico strategies, optimisation of genetic promoters, programmable genetic controls, modular vector systems, complementation systems and CRISPRi-mediated metabolic engineering.
About the authors
Dr Payel Datta is Research Scientist at the Rensselaer Polytechnic Institute, The Center for Biotechnology and Interdisciplinary Studies (CBIS).
References
- DeAngelis PL. Heparosan, a promising ‘naturally good’ polymeric conjugating vehicle for delivery of injectable therapeutics. Expert Opin Drug Deliv. 2015 Mar 4 [cited 2020 Oct 30];12(3):349–52. Available from: http://www.tandfonline.com…
- Cress BF, Erkert KA, Barquera B, Koffas MAG. Draft genome sequence of Escherichia coli strain ATCC 23506 (serovar O10:K5:H4). Genome Announc. 2013;1:(2):e0004913.
- Xu Y, Chandarajoti K, Zhang X, Pagadala V, Dou W, Hoppensteadt DM, et al. Synthetic oligosaccharides can replace animal-sourced low–molecular weight heparins. Sci Transl Med. 2017 Sep 6 [cited 2020 Oct 30];9(406). Available from: http://stm.sciencemag.org/
- Kwon PS, Oh H, Kwon SJ, Jin W, Zhang F, Fraser K, et al. Sulfated polysaccharides effectively inhibit SARS-CoV-2 in vitro. Vol. 6, Cell Discovery. Springer Nature; 2020 [cited 2020 Oct 30]. p. 50. Available from: https://doi.org/10.1038/s41421-020-00192-8
- Wang Z, Linhardt RJ, Dordick JS, Bhaskar U. K5 heparosan fermentation and purification. US Pat #8,883,452. 2014 [cited 2020 Jul 28]; Available from: https://patents.google.com/patent/US8883452B2/en
- de Oliveira JD, Carvalho LS, Gomes AMV, Queiroz LR, Magalhães BS, Parachin NS. Genetic basis for hyper production of hyaluronic acid in natural and engineered microorganisms. Microb Cell Fact. 2016 Jul 1 [cited 2020 Nov 2];15(1):119. Available from: http://microbialcellfactories.biomedcentral.com…
- https://www.fda.gov/media/100238/download. [cited 2020 Oct 30]. Available from: https://www.fda.gov/media/100238/download
- Linhardt RJ, Liu J. Synthetic heparin. Vol. 12, Current Opinion in Pharmacology. NIH Public Access; 2012 [cited 2020 Oct 30]. p. 217–9. Available from: https://www.ncbi.nlm.nih.gov/pmc/articles/PMC3496756/
- Qiao M, Lin L, Xia K, Li J, Zhang X, Linhardt RJ. Recent advances in biotechnology for heparin and heparan sulfate analysis. Talanta. 2020 Nov 1;219:121270.
- Kim SY, Jin W, Sood A, Montgomery DW, Grant OC, Fuster MM, et al. Characterization of heparin and severe acute respiratory syndrome-related coronavirus 2 (SARS-CoV-2) spike glycoprotein binding interactions. Antiviral Res. 2020 Sep 1;181:104873.
- Baytas SN, Linhardt RJ. Advances in the preparation and synthesis of heparin and related products. Drug Discov Today. 2020 Sep 16;
- Cress BF, Toparlak OD, Guleria S, Lebovich M, Stieglitz JT, Englaender JA, et al. CRISPathBrick: Modular combinatorial assembly of type II-A CRISPR arrays for dCas9-mediated multiplex transcriptional repression in E. coli. ACS Synth Biol [Internet]. 2015 Mar 30 [cited 2020 Oct 30];4(9):987–1000. Available from: https://pubs.acs.org/doi/abs/10.1021/acssynbio.5b00012
- EP2831237A1 – High molecular weight heparosan polymers and methods of production and use thereof. [cited 2020 Oct 30]. Available from: https://patents.google.com/patent/EP2831237A1/en
- US20160201103A1 – Heparosan-producing bacterium and heparosan manufacturing method. [cited 2020 Oct 30]. Available from: https://patents.google.com/patent/US20160201103A1/en
- Jones JA, Vernacchio VR, Lachance DM, Lebovich M, Fu L, Shirke AN, et al. EPathOptimize: A combinatorial approach for transcriptional balancing of metabolic pathways. Sci Rep. 2015 Jun 11 [cited 2020 Nov 2];5. Available from: https://pubmed.ncbi.nlm.nih.gov/26062452/
- Xu P, Rizzoni EA, Sul SY, Stephanopoulos G. Improving metabolic pathway efficiency by statistical model-based multivariate regulatory metabolic engineering. ACS Synth Biol. 2017;6(1):148–58.
Related topics
Biopharmaceuticals, Biotherapeutics, Cell Cultures, In Vitro, In Vivo
Related conditions
Cancer, Cardiovascular disease
Related organisations
US Food and Drug Administration (FDA)